Uranium and Depleted Uranium
(Updated December 2009)
http://www.world-nuclear.org/info/Nuclear-Fuel-Cycle/Uranium-Resources/Uranium-and-Depleted-Uranium/#.UhXJX3-N6So
- The basic fuel for a nuclear power reactor is uranium – a heavy metal able to release abundant concentrated energy.
- Uranium occurs naturally in the Earth's crust and is mildly radioactive.
- Depleted uranium is a by-product from uranium enrichment.
- The health hazards associated with any uranium are much the same as those for lead.
The Earth's uranium (chemical symbol U) was apparently formed in
supernovae up to about 6.6 billion years ago (see information page on The Cosmic Origins of Uranium).
Its radioactive decay provides the main source of heat inside the
Earth, causing convection and continental drift. As decay proceeds, the
final product, lead, increases in relative abundance.
Uranium was discovered by Martin Klaproth, a German chemist, in 1789
in the mineral pitchblende, and was named after the planet Uranus. It
occurs in most rocks in concentrations of 2 to 4 parts per million and
is as common in the Earth's crust as tin, tungsten and molybdenum and
about 40 times as common as silver. It is also found in the oceans, at
an average concentration of 1.3 parts per billion. There are a number of
locations in different parts of the world where it occurs in
economically-recoverable concentrations. When mined, it yields a mixed
uranium oxide product, U3O8. Uraninite, or pitchblende, is the most common uranium mineral.
In the past, uranium was also used to colour glass (from as early as
79 AD) and deposits were once mined in order to obtain its decay
product, radium. This element was used in luminous paint, particularly
on the dials of watches and aircraft instruments up to the 1950s, and in
medicine for the treatment of disease.
For many years from the 1940s, virtually all of the uranium that was
mined was used in the production of nuclear weapons, but this ceased to
be the case in the 1970s. Today the only substantial use for uranium is
as fuel in nuclear reactors, mostly for electricity generation.
Uranium-235 is the only naturally-occurring material which can sustain a
fission chain reaction, releasing large amounts of energy.
While nuclear power is the predominant use of uranium, heat from
nuclear fission can be used for industrial processes. It is also used
for marine propulsion (mostly naval). And small nuclear reactors are
important for making radioisotopes.
The uranium atom
Uranium is one of the heaviest of all the naturally-occurring
elements and has a specific gravity of 18.7. Its melting point is
1132ºC.a
Like other elements, uranium occurs in slightly differing forms known
as isotopes. These isotopes differ from each other in the number of
neutron particles in the nucleus. Natural uranium as found in the
Earth's crust is a mixture of three isotopes: uranium-238 (U-238),
accounting for 99.275%; U-235 – 0.720%; and traces of U-234 – 0.005%.
The isotope U-235 is important because under certain conditions it
can readily be split, yielding a lot of energy. It is therefore said to
be 'fissile'. Meanwhile, like all radioactive isotopes, it decays. U-238
decays very slowly, its half-lifeb
being the same as the age of the Earth. This means that it is barely
radioactive, less so than many other isotopes in rocks and sand.
Uranium-238 has a specific radioactivity of 12.4 kBq/g, and U-235 80
kBq/g, but the smaller amount of U-234 is very active (231 MBq/g) so the
specific radioactivity of natural uranium (25 kBq/g) is about double
that of U-238 despite it consisting of over 99% U-238.c In decay it generates 0.1 watts/tonne and this is enough to warm the Earth's mantle.
Uranium fission
The nucleus of the U-235 isotope comprises 92 protons and 143
neutrons (92 + 143 = 235). When the nucleus of a U-235 atom is split in
two by a neutrond,
some energy is released in the form of heat, and two or three
additional neutrons are thrown off. If enough of these expelled neutrons
split the nuclei of other U-235 atoms, releasing further neutrons, a
chain reaction can be achieved. When this happens over and over again,
many millions of times, a very large amount of heat is produced from a
relatively small amount of uranium.
It is this process, in effect 'burning' uranium, which occurs in a
nuclear reactor. In a nuclear reactor the uranium fuel is assembled in
such a way that a controlled fission chain reaction can be achieved. The
heat created by splitting the U-235 atoms is then used to make steam
which spins a turbine to drive a generator, producing electricity.
Whereas the U-235 atom is 'fissile', the U-238 atom is said to be
'fertile'. This means that it can capture a neutron and become
(indirectly) plutonium-239, which is fissile. Pu-239 is very much like
U-235, in that it can fission following neutron capture, also yielding a
lot of energye.
Because there is so much U-238 in a reactor core (most of the fuel),
these reactions occur frequently, and in fact about one-third of the
energy yield typically comes from burning Pu-239.
Both uranium and plutonium were used to make bombs before they became
important for making electricity and radioisotopes. But the type of
uranium and plutonium for bombs is different from that in a nuclear
power plant. Bomb-grade uranium is highly enriched (>90% U-235,
instead of about 3.5-5.0% in a power plant); bomb-grade plutonium is
fairly pure (>90%) Pu-239 and is made in special reactors.
Uranium as a fuel for nuclear power
About 14% of the world's electricity is generated from uranium in nuclear reactors1. This amounts to over 2700 billion kWh, as much as from all sources worldwide in 1988.2
It comes from about 435 nuclear reactors with a total output capacity
of about 370,000 MWe operating in 30 countries. Over 50 more reactors
are under construction and another 430 are on the drawing board3.
A typical 1000 megawatt (MWe) reactor can provide enough electricity
for a modern city of close to one million people, about 7 billion kWh
per year.
Belgium, Bulgaria, Czech Republic, Finland, France, Hungary, South
Korea, Lithuania, Slovakia, Slovenia, Sweden, Switzerland and Ukraine
all get 30% or more of their electricity from nuclear reactors. Germany
and Japan derive more than a quarter of their electricity from uranium.
The USA has over 100 reactors operating, supplying 20% of its
electricity4.
Nuclear power stations and fossil-fuelled power stations of similar
capacity have many features in common. Both require heat to produce
steam to drive turbines and generators. In a nuclear power station,
however, the fissioning of uranium atoms replaces the burning of coal or
gas. The chain reaction that takes place in the core of a nuclear
reactor is controlled by rods which absorb neutrons. They are inserted
or withdrawn to set the reactor at the required power level. The fuel
elements are surrounded by a substance called a moderator to slow the
speed of the emitted neutrons and thus enable the chain reaction to
continuef. Water, graphite and heavy water are used as moderators in different types of reactors.
Sources of uranium
Uranium is widespread in many rocks, and even in seawater. However,
like other metals, it is seldom sufficiently concentrated to be
economically recoverable. Where it is, we speak of an orebody. In
defining what is ore, assumptions are made about the cost of mining and
the market price of the metal. Uranium resources are therefore
calculated as tonnes recoverable up to a certain cost.
Australia's uranium resources are about 25% of the world's total, but
Canada has been the world's leading producer to 2008, then being
overtaken by Kazakhstang.
Other countries with known resources include Russian Federation, USA,
South Africa, Namibia, Niger, Brazil and Ukraine. Many more countries
have smaller deposits which could be mined. (See information page on Supply of Uranium).
Uranium is sold only to countries which are signatories of the
Nuclear Non-Proliferation Treaty, and which allow international
inspection to verify that it is used only for peaceful purposes.
From uranium ore to reactor fuel
Uranium ore can be mined by underground or open-cut methods,
depending on its depth. After mining, the ore is crushed and ground up.
Then it is treated with acid to dissolve the uranium, which is then
recovered from solution. Uranium may also be mined by in situ leaching (ISL), where it is dissolved from the orebody in situ and pumped to the surface.
The end product of the mining and milling stages, or ISL, is uranium oxide concentrate (U3O8).
Before it can be used in a reactor for electricity generation, however,
it must undergo a series of processes to produce a useable fuel.
For most of the world's reactors, the next step in making a useable
fuel is to convert the uranium oxide into a gas, uranium hexafluoride
(UF6), which enables it to be enrichedh. Enrichment increases the proportion of the U-235 isotope from its natural level of 0.7% to 3-5% (see information page on Uranium Enrichment).
This enables greater technical efficiency in reactor design and
operation, particularly in larger reactors, and allows the use of
ordinary water as a moderator. A by-product (sometimes considered a
waste product) of enrichment is depleted uranium (about 86% of the
original feed).
After enrichment, the UF6 gas is converted to uranium dioxide (UO2)
which is formed into fuel pellets. These fuel pellets are placed inside
thin metal tubes which are assembled in bundles to become the fuel
elements for the core of the reactor. UO2 has a very high melting point – 2865°C (compared with uranium metal – 1132°C).
Used reactor fuel is removed from the reactor and stored, either to be reprocessed or disposed of underground.
The uranium orebody contains both U-235 and (mostly) U-238. About 95%
of the radioactivity in the ore is from the U-238 decay series. This
has 14 radioactive isotopes in secular equilibrium, thus each represents
7% of the total. (In the case of Ranger ore - with 0.3% U308 - it has
about 450 kBq/kg, so irrespective of the mass proportion, 32 kBq/kg per
nuclide in that decay series.) When the ore is processed, the U-238 and
the very much smaller masses of U-234 (and the U-235) are removed. The
balance becomes tailings, and at this point has about 86% of its
original intrinsic radioactivity. However, with the removal of most
U-238, the following two short-lived decay products (Th-234 &
Pa-234) soon disappear, leaving the tailings with a little over 70% of
the radio-activity of the original ore after several months. The
controlling long-lived isotope then becomes Th-230 which decays with a
half life of 77,000 years to radium-226 followed by radon-222.
Recycled (reprocessed) uranium
Uranium comprises about 96% of used fuel. When used fuel is reprocessed, both plutonium and uranium are recovered separately.
Uranium recovered from reprocessing used nuclear fuel is mostly U-238
with about 1% U-235, so it needs to be converted and re-enriched. This
is complicated by the presence of impuritiesi
and two isotopes in particular, U-232 and U-236, which are formed by or
following neutron capture in the reactor, and increase with higher
burn-up levelsj.
U-232 is largely a decay product of Pu-236, and increases with storage
time in used fuel, peaking at about ten years. Both U-232 and U-236
decay much more rapidly than U-235 and U-238, and one of the daughter
products of U-232 emits very strong gamma radiation, which means that
shielding is necessary in any plant handling material with more than
very small traces of it. U-236, comprising about 0.5% of recovered
uranium, is a neutron absorber which impedes the chain reaction, and
means that a higher level of U-235 enrichment is required in the product
to compensate.
Because they are lighter than U-238, both U-232 and U-236 tend to
concentrate in the enriched (rather than depleted) output, so
reprocessed uranium (RepU) that is re-enriched for fuel must be
segregated from enriched fresh uranium. Enriched RepU has an activity of
over 250 kBq/g, which compares with 82kBq/g (most of this being from
U-234) for enriched fresh uranium. The presence of U-236 in particular
means that most reprocessed uranium can normally be recycled only once.
In the future, laser enrichment techniques may be able to remove these
difficult isotopes.
Uranium from thorium
Thorium, as well as uranium, can be used as a nuclear fuel. Although
not fissile itself, Th-232 will absorb slow neutrons to produce
uranium-233 (U-233)k,
which is fissile (and long-lived). The irradiated fuel can then be
unloaded from the reactor, the U-233 separated from the thorium, and fed
back into another reactor as part of a closed fuel cycle.
U-233 has higher neutron yield per neutron absorbed than U-235 or
Pu-239. Given a start with some other fissile material (U-233, U-235 or
Pu-239) as a driver, a breeding cycle similar to but more efficient than
that with U-238 and plutonium (in conventional thermal neutron
reactors) can be set up. The driver fuels provide all the neutrons
initially, but are progressively supplemented by U-233 as it forms from
the thorium. However, the intermediate product protactinium-233 (Pa-233)
is a neutron absorber which diminishes U-233 yield. (See information
page on Thorium).
Other uses of uranium-fuelled reactors
There are also other uses for uranium-fuelled nuclear reactors. Over
200 small nuclear reactors power some 150 ships, mostly submarines, but
ranging from icebreakers to aircraft carriers. These can stay at sea for
very long periods without having to make refuelling stops. In most such
vessels the steam drives a turbine directly geared to propulsion.
The heat produced by nuclear reactors can also be used directly
rather than for generating electricity. In Russia, for example, it is
used to heat buildings and elsewhere it provides heat for a variety of
industrial processes such as water desalination. In the future,
high-temperature reactors could be used for industrial processes such as
thermochemical production of hydrogen.
Radioisotope production in uranium fuelled reactors
Radioactive materials (radioisotopes) play a key role in the
technologies that provide us with food, water and good health and have
become a vital part of modern life. They are produced by bombarding
small amounts of particular elements with neutrons. Using relatively
small special purpose nuclear reactors (usually called research
reactors), a wide range of radioisotopes can be made at low cost. The
use of radioisotopes has become widespread since the early 1950s, and
there are now some 280 research reactors in 56 countries producing them.
In medicine, radioisotopes are widely used for diagnosis, and also
for treatment and research. Radioactive chemical tracers emit gamma
radiation which provides diagnostic information about a person's anatomy
and the functioning of specific organs. Radiotherapy also employs
radioisotopes in the treatment of some illnesses, such as cancer. More
powerful gamma sources are used to sterilise syringes, bandages and
other medical equipment. About one in two people in Western countries is
likely to experience the benefits of nuclear medicine in their
lifetime, and gamma sterilisation of equipment is almost universal. (See
information page on Radioisotopes in Medicine.)
In the preservation of food, radioisotopes are used to inhibit the
sprouting of root crops after harvesting, to kill parasites and pests,
and to control the ripening of stored fruit and vegetables. Irradiated
foodstuffs are accepted by world and national health authorities for
human consumption in an increasing number of countries. They include
potatoes, onions, dried and fresh fruits, grain and grain products,
poultry and some fish. Some prepacked foods can also be irradiated.
Agriculturally, in the growing crops and breeding livestock,
radioisotopes also play an important role. They are used to produce
high-yielding, disease- and weather-resistant varieties of crops, to
study how fertilisers and insecticides work, and to improve the
productivity and health of domestic animals. Industrially, and in
mining, they are used to examine welds, to detect leaks, to study the
rate of wear of metals, and for on-stream analysis of a wide range of
minerals and fuels. (See information page on Radioisotopes in Industry.)
Environmentally, radioisotopes are used to trace and analyse
pollutants, to study the movement of surface water, and to measure water
runoffs from rain and snow, as well as the flow rates of streams and
rivers.
Most household smoke detectors use a radioisotope (americium-241)
derived from the plutonium formed in nuclear reactors. These alarms save
many lives. (See information page on Smoke Detectors and Americium.)
Depleted uranium
Every tonne of natural uranium produced and enriched for use in a
nuclear reactor gives about 130 kg of enriched fuel (3.5% or more
U-235). The balance is depleted uranium tails (U-238, typically with
0.25-0.30% U-235). This major portion has been depleted in its fissile
U-235 isotope (and, incidentally, U-234) by the enrichment process. It
is commonly known as DU if the focus is on the actual material, or tails
if the focus is on its place in the fuel cycle and its U-235 assay.
DU tails are either stored as UF6 or (especially in France) de-converted back to U3O8,
which is more benign chemically and thus more suited for long-term
storage. It is also less chemically toxic. Every year over 50,000 tonnes
of depleted uranium joins already substantial stockpiles in the USA,
Europe and Russia. World stock is about 1.5 million tonnes.
Some DU is drawn from these stockpiles to dilute high-enriched
(>90%) uranium released from weapons programs, particularly in
Russia, and destined for use in civil reactors (see information page on Military Warheads as a Source of Nuclear Fuel).
This weapons-grade material is diluted about 25:1 with depleted
uranium, or 29:1 with depleted uranium that has been enriched slightly
(to 1.5% U-235) to minimise levels of (natural) U-234 in the product.
Some, assaying 0.25-0.40% U-235, is sent to Russia for re-enrichment,
using surplus plant capacity there to produce either natural uranium
equivalent or low-enriched uranium (4-5% U-235).
Some DU is used for mixed oxide (MOX) fuel, by mixing with plutonium (see information page on Mixed Oxide (MOX) Fuel).
Other uses depend on the metal's very high density (1.7 times that of
lead). Hence, where maximum mass must fit in minimum space, such as
aircraft control surface and helicopter counterweights, yacht keels,
etc, it is often well suited. Until the mid 1970s it was used in dental
porcelains. In addition it is used for radiation shielding, being some
five times more effective than lead in this role.
Also because of its density, it is used as solid slugs or penetrators
in armour-piercing projectiles, alloyed with abut 0.75% titanium. DU is
pyrophoric, so that upon impact about 30% of the projectile atomises
and burns to uranium oxide dust. It was widely used in the 1990/91 Gulf
War (300 tonnes) and less so in the 1998/99 Kosovo War (11 tonnes).
Health aspects of DU
Depleted uranium is not classified as a dangerous substance
radiologically, though it is a potential hazard in large quantities,
beyond what could conceivably be breathed. Its emissions are very low,
since the half-life of U-238 is the same as the age of the Earth (4.5
billion years). There are no reputable reports of cancer or other
negative health effects from radiation exposure to ingested or inhaled
natural or depleted uranium, despite much study.
However, uranium does have a chemical toxicity about the same as that
of lead, so inhaled fume or ingested oxide is considered a health
hazard. Most uranium actually absorbed into the body is excreted within
days, the balance being laid down in bone and kidneys. Its biological
effect is principally kidney damage. The World Health Organization (WHO)
has set a tolerable daily intake level for uranium of 0.6 microgram/kg
body weight, orally. (This is about eight times our normal background
intake from natural sources.) Standards for drinking water and
concentrations in air are set accordingly.
Like most radionuclides, it is not known as a carcinogen, or to cause birth defects (from effects in utero)
or to cause genetic mutations. Radiation from DU munitions depends on
how long since the uranium has been separated from the lighter isotopes
so that its decay products start to build up. Decay of U-238 gives rise
to Th-234, Pa-234 (beta emitters) and U-234 (an alpha emitter)m.
On this basis, in a few months, DU is weakly radioactive with an
activity of around 40 kBq/g quoted. (If it is fresh from the enrichment
plant and hence fairly pure, the activity is 15 kBq/g, compared with 25
kBq/g for pure natural uranium. Fresh DU from enriching reprocessed
uranium has U-236 in it and more U-234 so is about 23 kBq/g.)
In
2001, the UN Environment Programme (UNEP) examined the effects of nine
tonnes of DU munitions having been used in Kosovo, checking the sites
targeted by it5.
UNEP found no widespread contamination, no sign of contamination in
water of the food chain and no correlation with reported ill-health in
NATO peacekeepers. A two-year study6 by Sandia National Laboratories in USA reported in 2005 that consistent with earlier studiesn,
reports of serious health risks from DU exposure during the 1991 Gulf
War are not supported by medical statistics or by analysis.
An editorial in the Radiological Protection Bulletin of the UK's National Radiation Protection Board stated: "DU
is radioactive and doses from inhalation of dust or from handling bare
spent rounds need to be assessed properly. However, the scientific
consensus at present is that the risks are likely to be small and easily
avoidable, especially compared with the other risks the armed forces
have to take in war."8
Thus DU is clearly dangerous for military targets, but for anyone
else – even in a war zone – there is little hazard. Ingestion or
inhalation of uranium oxide dust resulting from the impact of DU
munitions on their targets is the main possible exposure route.
Further Information
a. See also Webelements' uranium webpage [Back]
b. The half-life is the time it takes for a radionuclide to lose half of its own radioactivity. [Back]
c. The becquerel (Bq) is a unit or measure of actual radioactivity in
material (as distinct from the radiation it emits), with reference to
the number of nuclear disintegrations per second (1 Bq = 1
disintegration/sec). For further information on units of radioactivity
see the Units of radiation and radioactivity section in the information page on Radiation and Nuclear Energy [Back]
d. U-235 can fission following capture of a low-energy (or 'thermal')
neutron to form a new compound nucleus, which then splits into two
daughter fragments and two or three neutrons (average around 2.5),
releasing energy in the process. For further information on nuclear
fission, see WNA's Some Physics of Uranium education paper [Back]
e. Sometimes Pu-239 simply captures a neutron without splitting, and
it becomes Pu-240. Because the Pu-239 is either progressively burned or
becomes Pu-240, the longer the fuel stays in the reactor the more Pu-240
accumulates in it. The significance of this is that when the used fuel
is removed after about three years, the plutonium in it is not suitable
for making weapons – because Pu-240 has a relatively high rate of
spontaneous fission – but can be recycled as fuel (see also information
page on Plutonium). [Back]
f. Neutrons released in fission are initially fast (velocity about 109 cm/sec, or energy above 1 MeV), but fission in U-235 is most readily caused by slow (thermal) neutrons (velocity about 105
cm/sec, or energy about 0.02 eV). A moderator material comprising light
atoms thus surrounds the fuel rods in a reactor to slow down the
neutrons in elastic collisions. For further information, see WNA's
education paper on Some Physics of Uranium [Back]
g. Kazakhstan is now reported to be the world's leading uranium producer – see Kazakhstan takes top spot in 2009, World Nuclear News (5 January 2010) [Back]
h. For reactors which use natural uranium as their fuel (and which require graphite or heavy water as a moderator) the U3O8 concentrate simply needs to be refined and converted directly to uranium dioxide. [Back]
i. Recovered uranium (especially from earlier military reprocessing)
may be contaminated with traces of fission products. Over 2002-06 USEC
cleaned up 7400 tonnes of technetium-contaminated uranium from the US
Department of Energy. [Back]
j. Recovered uranium also contains a higher proportion of U-234 than
fresh reactor fuel. As well as having a greater specific activity than
both U-235 and U-238, the presence of U-234 alters the reactivity as it
absorbs neutrons. [Back]
k.
Neutron absorption by Th-232 produces Th-233, which has a half-life of
about 22 minutes. This undergoes beta decay to form Pa-233 (half-life 27
days), most of which forms U-233 by further beta decay. Around 11% of
the U-233 is converted by further neutron absorption to U-235, which is
the fissile isotope of uranium used in conventional nuclear reactors. A
small amount of the Pa-233 and U-233 forms U-232 in the reactor.
Separated U-233 is therefore always contaminated with traces of U-232,
which has a 69-year half-life but whose daughter productsl,
particularly thallium-208, are strong gamma emitters with very short
half-lives. This creates significant problems in handling the bred U-233
and makes it easy to detect, hence conferring proliferation resistance.
[Back]
l. The decay chain of U-232 has six short-lived decay products before Tl-208, which precedes stable Pb-208. [Back]
m. U-238 (half-life 4.5 billion years) decays to thorium-234
(half-life 24 days), which beta decays to protactinium-234 (half-life
one minute), which beta decays to U-234 (alpha emitter, half-life
246,000 years). [Back]
n. For example, a 2001 paper by the Australasian Radiation Protection Society (ARPS)7,
which quotes several studies, concludes that health risks associated
with the levels of DU exposure experienced during the Gulf War are
essentially zero. A summary of the ARPS statement reads as follows:
Some military personnel involved in the 1991 Gulf War complained
of continuing stress-like symptoms for which no obvious cause was found.
These symptoms were at times attributed to the use of depleted uranium
in shells and other missiles, which are said to have caused toxic
effects. Similar complaints arose from later fighting in the Balkans
(Kosovo). Because of the latency period for the induction of cancer by
radiation, it is not credible that any cases of radiation-induced cancer
could in the short term be attributed to the Kosovo conflict.
Furthermore, extensive studies have concluded that no radiological
health hazard should be expected from exposure to depleted uranium. The
risk from external exposure is essentially zero, even when pure metal is
handled. No detectable increases of cancer, leukaemia, birth defects or
other negative health effects have ever been observed from radiation
exposure to inhaled or ingested natural uranium concentrates, at levels
far exceeding those likely in areas where DU munitions have been used.
This is mainly because the low radioactivity per unit mass of uranium
means that the mass needed for significant internal exposure would be
virtually impossible to accumulate in the body – and DU is less than
half as radioactive as natural uranium. [Back]
1. Key World Energy Statistics 2009, OECD International Energy Agency, 9 rue de la Fédération, 75739 Paris Cedex 15, France (2009) [Back]
2. Table 8.2a of Annual Energy Review 2008, U.S. Energy Information Administration, Report No. DOE/EIA-0384(2008) [Back]
3. World Nuclear Association table of World Nuclear Power Reactors & Uranium Requirements [Back]
4. World Nuclear Association table of Nuclear share figures [Back]
5. Depleted Uranium in Kosovo: Post-Conflict Environmental Assessment United Nations Environment Programme (2001) [Back]
6. Albert C. Marshall, An Analysis of Uranium Dispersal and Health Effects Using a Gulf War Case Study, Sandia National Laboratories, USA, SAND2005-4331 (July 2005) [Back]
7. Potential Health Effects of Depleted Uranium in Munitions, Australasian Radiation Protection Society media release (8 February 2001) [Back]
8. Michael Clark, Editorial, Radiological Protection Bulletin No. 229, P3 (March 2001), National Radiological Protection Board [Back]
General sources
World Health Organization fact sheet on depleted uranium
US Department of Energy Depleted UF6 Management Information Network website (http://web.ead.anl.gov/uranium/)
Radiation Protection Bulletin No. 167, P.13-16 (July 1995), National Radiological Protection Board
Balkans Task Force Final Report, The Kosovo Conflict – Consequences for the Environment & Human Settlements, United Nations Environment Programme and United Nations Centre for Human Settlements (Habitat), ISBN 9280718011 (1999)
Management of Depleted Uranium, OECD Nuclear Energy Agency, OECD Publishing, ISBN 9789264195257 (Aug 2001)
Bulletin of the Atomic Scientists, Volume 55, Number 6, (November-December 1999)
The Cosmic Origins of Uranium
(November 2006)
- Uranium drives 16% of our electricity worldwide, yet this fact pales into insignificance when we consider the role uranium has played in the evolution of the Earth.
- The Earth's uranium was produced in one or more supernovae over 6 billion years ago.
- Uranium later became enriched in the continental crust.
Geologists and geochemists have been studying the abundance,
distribution and chronometric potential of the isotopes of uranium for
more than a century. Their work stems from Klaproth's discovery in 1789
of the heaviest naturally occurring element, Becquerel's demonstration
in 1896 that uranium salts are radioactive, Boltwood's conclusion in
1905 that lead as well as helium is a decay product of uranium, and
Rutherford's suggestion in 1906 of the geological time-keeping potential
of radioactivity. From a geochemical point of view, some of the major
questions are:
- Where did the uranium now in the Earth come from?
- What effects has the comparatively trivial uranium content of the Earth had on the evolution of the planet and, conversely, are there feedbacks controlling the geochemical cycle of uranium that vary secularly (i.e. over long, indefinite periods of time)?
- Can we track through time the way uranium has been recycled through the exosphere, crust and mantle of the Earth?

Cosmic abundance of elements
For many years, since the 1930s, a large number of scientists have
been occupied with determining the abundances of the elements and their
isotopes in the objects comprising the solar system, and with accounting
for the abundance patterns observed. In fact, spectroscopic
measurements show that the abundances of elements in stars vary and that
there is no single applicable 'cosmic abundance' pattern.
Closer to home, there are major differences in abundances of the
elements in the various planets that orbit our hydrogen-helium dominated
Sun. The terrestrial planets, including the Earth, are relatively
depleted in the potentially gaseous or volatile elements (hydrogen,
helium, carbon and neon) and are dominated by elements of low and even
atomic number (oxygen, magnesium, silicon and iron). On this scale,
uranium - the abundance of which in the Sun is only 10 -12
that of hydrogen - is an exceedingly rare element. Furthermore,
measurements of oxygen isotopes in meteorites show that the solar system
as a whole is not homogeneous in terms of isotopic ratios. All these
variations point to a conclusion that multiple sources were involved in
the production of proto-solar system material.
Where did uranium come from?
Cosmochemists have been concerned not only with patterns and secular
trends of abundance of the elements in galaxies but also with the
origins of abundance anomalies in particular stars and with theories on
the synthesis of different nuclei to account for these observations.
According to the theories developed, the Earth's uranium was produced in
one or more supernovae ("An explosive brightening of a star in which
the energy radiated by it increases by a factor of ten billion ... A
supernova explosion occurs when a star has burned up all its available
nuclear fuel and the core collapses catastrophically." - Oxford
Dictionary of Physics). The main process concerned was the rapid capture
of neutrons on seed nuclei at rates greater than disintegration through
radioactivity. The neutron fluxes required are believed to occur during
the catastrophically explosive stellar events called supernovae.
Gravitational compression of iron (the island of nuclear stability,
incapable of further exothermic fusion reactions) and sudden collapse in
the centre of a massive star triggers the explosive ejection of much of
the star into space, together with a flood of neutrons. Remnants of
hundreds of supernovae have been found, and we "witnessed" one in the
Magellanic Clouds in 1987.
So, we know that the Earth's uranium was produced through this
process in one or more supernovae, and that this material was inherited
by the solar system of which the Earth is a part.
We might further ask how long ago this synthesis of uranium occurred. Given
- the present day abundances of U-235 and U-238 in the various 'shells' forming our planet,
- a knowledge of the half-lives of these isotopes, and
- the age of the Earth (c 4.55 billion years) - known from various radiometric 'clocks', including those of the uranium-to-lead decay chains.
We can calculate the abundances of U-235 and U-238 at the time the
Earth was formed. Knowing further that the production ratio of U-235 to
U-238 in a supernova is about 1.65, we can calculate that if all of the
uranium now in the solar system were made in a single supernova, this
event must have occurred some 6.5 billion years ago.
This 'single stage' is, however, an oversimplification. In fact,
multiple supernovae from over 6 billion to about 200 million years ago
were involved. Additionally, studies of the isotopic abundances of
elements, such as silicon and carbon in meteorites, have shown that more
than ten separate stellar sources were involved in the genesis of solar
system material. Thus the relative abundance of U-235 and U-238 at the
time of formation of the solar system:
- cannot be inverted to a 'single stage' model age,
- is essentially an accidental and unique value, and
- reflects the input of the explosive debris of many progenitor stars.
Enrichment in Earth's crust
Many analyses have been made of the uranium in the rocks forming the
continental and oceanic crusts, and in samples of the Earth's mantle
exposed as uplifted slices in mountain belts or as 'xenoliths' in
basalts and kimberlites (hosts of diamonds).
We can have some confidence that these measurements are robust for
the crust and upper mantle of the Earth, but less confidence that we
know the abundance of uranium in the lower mantle and the outer and
inner cores. While on average the abundance of uranium in meteorites is
about 0.008 parts per million (gram/tonne), the abundance of uranium in
the Earth's 'primitive mantle' - prior to the extraction of the
continental crust - is 0.021 ppm. Allowing for the extraction of a
core-forming iron-nickel alloy with no uranium (because of the
characteristic of uranium which makes it combine more readily with
minerals in crustal rocks rather than iron-rich ones), this still
represents a roughly twofold enrichment in the materials forming the
proto-Earth compared with average meteoritic materials.
The present-day abundance of uranium in the 'depleted' mantle exposed
on the ocean floor is about 0.004 ppm. The continental crust, on the
other hand, is relatively enriched in uranium at some 1.4 ppm. This
represents a 70-fold enrichment compared with the primitive mantle. In
fact, the uranium lost from the 'depleted' oceanic mantle is mostly
sequestered in the continental crust.
It is likely that the process or processes which transferred uranium
from the mantle to the continental crust are complex and multi-step.
However, for at least the past 2 billion years they have involved:
- formation of oceanic crust and lithosphere through melting of the mantle at mid-ocean ridges,
- migration of this oceanic lithosphere laterally to a site of plate consumption (this is marked at the surface by a deep-sea trench),
- production of fluids and magmas from the downgoing (subducted) lithospheric plate and overriding mantle 'wedge' in these subduction zones,
- transfer of these fluids/melts to the surface in zones of 'island arcs' (such as the Pacific's Ring of Fire),
- production of continental crust from these island arc protoliths, through remelting, granite formation and intra-crustal recycling.
All through this crust-forming cycle, the lithophile character of
uranium is manifest in the constancy of the potassium to uranium ratio
at about 10,000 in the rock range from peridotite to granite. Because we
would like to keep track of how uranium is distributed in the Earth,
the abundance and isotopic characteristics of lead - the radiogenic
daughter of U-235 and U-238 - are useful parameters. Table 1
below highlights the relatively low abundance of lead in the Earth's
mantle and the consequent high uranium to lead ratio, compared with
meteorites. The difference in abundance can most likely be explained by
lead's volatile nature and tendency to combine with iron, with lead
being lost during terrestrial accretion and core separation. One of the
consequences, of course, of these high ratios is the comparatively high
radiogenic/non-radiogenic content of Pb-207/Pb-204 and Pb-206/Pb-204 in
the Earth's crust and mantle compared with meteorites or the earth's
core. (Pb-207 is the final stable decay product of U-235, and Pb-206 is
that of U-238. Pb-204 is non-radiogenic.
Table 1
U abundance (ppm) |
Pb abundance (ppm) |
U/Pb ratio | |
Meteorites | 0.008 | 2.470 | 0.003 |
---|---|---|---|
Primitive mantle | 0.021 | 0.185 | 0.113 |
Continental crust | 1.4 | 12.6 | 0.111 |
The figure given for the continental crust is an average of the
entire crust. Of course, local concentration of uranium can far exceed
these values, ranging up to 50 ppm disseminated in some granites, to
much higher values in ore deposits. In fact, in the geological past,
local concentrations of uranium have occasionally achieved natural
criticality, for example the Oklo reactors in Gabon.
Energy source
Convection in the outer core and the mantle, whereby heat is
transferred by movement of heated matter, governs many of the Earth's
endogenous processes.
The convection in the core may be driven by the heat released during
progressive solidification of the core (latent heat of crystallisation)
and leads to the self-sustaining terrestrial dynamo which is the source
of the Earth's magnetic field. Heat transfer from the core at the
core/mantle boundary is also believed to trigger upwellings of
relatively hot, and hence low density, plumes of material. These plumes
then ascend, essentially without gaining or losing heat, and undergo
decompression melting close to the Earth's surface at 'hot spots' like
Hawaii, Reunion and Samoa.
However, the primary source of energy driving the convection in the
mantle is the radioactive decay of uranium, thorium and potassium. In
the present Earth, most of the energy generated is from the decay of
U-238 (c 10-4 watt/kg). At the time of the Earth's formation,
however, decay of both U-235 and K-40 would have been subequal in
importance and both would have exceeded the heat production of U-238.
A simple way of viewing the process of plate tectonics - the
formation and disposal of oceanic lithosphere - is that this is the
mechanism by which the mantle sheds heat. Conversely, 'mantle plumes/hot
spots' are the way the core sheds heat. In terms of total heat loss
from the Earth at present, plate activity constitutes about 74%, hot
spots account for approximately 9% and radiogenic heat lost directly
from the continental crust is some 17%. The Earth is well insulated
thermally and the heat loss from the surface now can reflect heat
generation a considerable time in the past.
Measurements of heat have led to estimates that the Earth is
generating between 30 and 44 terawatts of heat, much of it from
radioactive decay. Measurements of antineutrinos have provisionally
suggested that about 24 TW arises from radioactive decay. Professor Bob
White provides the more recent figure of 17 TW from radioactive decay in
the mantle. This compares with 42-44 TW heat loss at the Earth's
surface from the deep Earth. The balance comes from changes in the core.
(There is very much greater heat loss arising from incident solar
radiation, which is quite distinct.)
Atmosphere and greenhouse effect, the role of plants
Apart from the fundamental physical and chemical differentiation of
the Earth driven by plate tectonics, lithosphere formation and
destruction are also critical for many processes in the outer layer of
the atmosphere. We know, for example, that during periods of enhanced
oceanic lithosphere formation, such as occurred during the Cretaceous
period some 100 million years ago, the mid-ocean ridges stood higher,
triggering flooding of the low-lying portions of the continents. In fact
the Laurasian part of the former Pangea supercontinent was drowned to a
greater extent than the Gondwana part, maybe reflecting some
deep-seated thermal/compositional contrast. The effects were manifold
and include:
- enhanced carbon dioxide release causing increased carbon dioxide content of the ocean and the atmosphere,
- diminished continental surface area leading to a reduction in the titration through weathering of atmospheric carbon dioxide,
- sustained high atmospheric carbon dioxide levels leading to an enhanced greenhouse effect and warmer climate.
Secular changes have taken place in several atmospheric processes,
including a change in composition, from relatively reducing to
astonishingly oxidising. The odd-looking "equation" for atmosphere
production is:
CO2 + H2 = N2 + O2
where the primary, volcanically degassed inputs to the atmosphere are
on the left, and the cumulative abundant components are on the right
hand side of the equation. Nitrogen is a trace volcanic emission, not
utilised to any great extent in surface processes - including the
trivial effect of organic life - and merely accumulates in the
atmosphere. The Earth's distance from the Sun, together with the
greenhouse feedback, allows surface temperatures to be generally
sustained within the condensation range for water. Carbon dioxide
dissolves in water and is also sequestered in calcite by inorganic and
organic precipitation as limestone.
The remarkable feature of our atmosphere is the presence of molecular
oxygen released through photosynthesis, the process by which green
plants manufacture their carbohydrates from atmospheric carbon dioxide
and water:
6CO2 + 6H2O = C6H12O6 + 6O2
Photosynthesis can be traced back in time to about 3.8 billion years.
For a while, the oxygen released was consumed through oxidation of
reduced ferrous compounds at the Earth's surface. Ultimately, the oxygen
started to accumulate in the atmosphere as free oxygen some 2.5 billion
years ago.
In addition to many other effects, the change in the redox character
of the exosphere led to a fundamental change in the way uranium was
transported in the weathering-erosion-deposition cycle. Whereas under
reduced conditions uranium is relatively insoluble and stable as
uraninite (UO2), under oxidising conditions it becomes soluble (U6+)
and readily transported. Since 2.5 billion years ago ore deposits of
uranium have been formed primarily where reduction of uranium-bearing
fluids was achieved, for example by bacteria or through contact with
graphitic shales.
Uranium distribution through time
The oxidising atmosphere also led to an increased concentration of
uranium in the oceans and thence via transport in recirculating
hydrothermal fluids to relative enrichment in the oceanic crust. The
enhanced uranium transport from the exosphere to the Earth's interior -
via subduction of oceanic lithosphere and the subsequent
rehomogenisation of this lithosphere into the Earth's mantle - has had a
significant effect on the present distribution of uranium in the Earth,
and may account for some curious inconsistencies in the isotopic
characteristics of the mantle. For example, whereas the time-integrated
Pb-208 (stable final decay product of Th-232)/Pb-206 values of mid-ocean
ridge basalts indicate mantle source values of Th/U of about 4, the
measured values of Th/U and systematics of short-lived Th-U
disequilibria indicate a ratio of about 2. It is likely that since about
2.5 billion years ago injections of uranium into the mantle have been
effective in the reduction of the thorium to uranium ratio on an (upper)
mantle-wide scale.
An additional net effect is the selective reinjection of uranium as
opposed to lead - which is mostly stripped out in subduction zones and
returned immediately to the crust - into the mantle. We know that
overall, most basalts are being produced from a mantle with an enhanced
uranium/lead ratio and with apparent 'future' ages, compared with the
lead isotopic ratios characteristic of a closed system, single stage
evolution of uranium/lead in the Earth. This feature is sometimes
referred to by geochemists as the 'lead paradox', and may in part relate
to the feedback influence of an oxidising, life-triggered exosphere on
the interior of the Earth.
Natural nuclear reactors in the Earth's crust
At Oklo in Gabon, West Africa, about 2 billion years ago, at least 17
natural nuclear reactors commenced operation in a rich deposit of
uranium ore. Each operated at about 20 kW thermal. At that time the
concentration of U-235 in all natural uranium was 3.7 percent instead of
0.7 percent as at present (U-235 decays much faster than U-238, whose
half-life is about the same as the age of this planet.).
These natural chain reactions, started spontaneously by the presence
of water acting as a moderator, continued for about two million years
before finally dying away. During this long reaction period about 5.4
tonnes of fission products as well as 1.5 tonnes of plutonium together
with other transuranic elements were generated in the orebody. The
initial radioactive products have long since decayed into stable
elements but study of the amount and location of these has shown that
there was little movement of radioactive wastes during and after the
nuclear reactions. Plutonium and the other transuranics remained
immobile.
Georeactor theory
A quite different view of the role of uranium in the Earth is the
theory that much of the uranium in the primordial planet sunk to the
core and has formed a core there, some 8 km across, which has been
fissioning ever since. The depletion of U-235 over geological time has
not terminated the reaction because this core is a fast reactor (not
requiring any moderator) which breeds plutonium-239 from the U-238. The
georeactor theory has relatively little supporting evidence, and is not
widely supported.
Sources:
This paper, apart from the last two sections, and an addition quantifying internal energy generation, is by Prof. Richard Arculus, Australian National University, and is used with the author's permission. It is based on a paper presented by Professor Arculus at the Uranium Institute Mid-Term Meeting in Adelaide on 17 April 1996.
New Scientist 7/8/04.
This paper, apart from the last two sections, and an addition quantifying internal energy generation, is by Prof. Richard Arculus, Australian National University, and is used with the author's permission. It is based on a paper presented by Professor Arculus at the Uranium Institute Mid-Term Meeting in Adelaide on 17 April 1996.
New Scientist 7/8/04.
Supply of Uranium
(updated August 2012)http://www.world-nuclear.org/info/Nuclear-Fuel-Cycle/Uranium-Resources/Supply-of-Uranium/#.UhXLY3-N6So
- Uranium is a relatively common metal, found in rocks and seawater. Economic concentrations of it are not uncommon.
- Its availability to supply world energy needs is great both geologically and because of the technology for its use.
- Quantities of mineral resources are greater than commonly perceived.
- The world's known uranium resources increased 15% in two years to 2007 due to increased mineral exploration.
Uranium is a relatively common element in the crust of the Earth
(very much more than in the mantle). It is a metal approximately as
common as tin or zinc, and it is a constituent of most rocks and even of
the sea. Some typical concentrations are: (ppm = parts per million).
Very high-grade ore (Canada) - 20% U | 200,000 ppm U |
---|---|
High-grade ore - 2% U, | 20,000 ppm U |
Low-grade ore - 0.1% U, | 1,000 ppm U |
Very low-grade ore* (Namibia) - 0.01% U | 100 ppm U |
Granite | 3-5 ppm U |
Sedimentary rock | 2-3 ppm U |
Earth's continental crust (av) | 2.8 ppm U |
Seawater | 0.003 ppm U |
* Where uranium is at low levels in rock or sands
(certainly less than 1000 ppm) it needs to be in a form which is easily
separated for those concentrations to be called "ore" - that is,
implying that the uranium can be recovered economically. This means
that it need to be in a mineral form that can easily be dissolved by
sulfuric acid or sodium carbonate leaching.
An orebody is, by definition, an occurrence of mineralisation from
which the metal is economically recoverable. It is therefore relative to
both costs of extraction and market prices. At present neither the
oceans nor any granites are orebodies, but conceivably either could
become so if prices were to rise sufficiently.
Measured resources of uranium, the amount known to be economically
recoverable from orebodies, are thus also relative to costs and prices.
They are also dependent on the intensity of past exploration effort, and
are basically a statement about what is known rather than what is there
in the Earth's crust - epistemology rather than geology. See Appendix 2
for mineral resource and reserve categories.
Changes in costs or prices, or further exploration, may alter
measured resource figures markedly. At ten times the current price,
seawater might become a potential source of vast amounts of uranium.
Thus, any predictions of the future availability of any mineral,
including uranium, which are based on current cost and price data and
current geological knowledge are likely to be extremely conservative.
From time to time concerns are raised that the known resources might
be insufficient when judged as a multiple of present rate of use. But
this is the Limits to Growth fallacy, a major intellectual blunder
recycled from the 1970s, which takes no account of the very limited
nature of the knowledge we have at any time of what is actually in the
Earth's crust. Our knowledge of geology is such that we can be confident
that identified resources of metal minerals are a small fraction of
what is there. Factors affecting the supply of resources are discussed
further and illustrated in the Appendix.
Uranium availability
With those major qualifications the following Table gives some idea
of our present knowledge of uranium resources. The total and several
country figures are lower than two years earlier due to economic
factors, notably inflation of production costs. It can be seen that
Australia has a substantial part (about 31 percent) of the world's
uranium, Kazakhstan 12 percent, and Canada and Russia 9 percent each.
Known Recoverable Resources of Uranium 2011
tonnes U | percentage of world | |
Australia |
1,661,000
|
31%
|
---|---|---|
Kazakhstan |
629,000
|
12%
|
Russia |
487,200
|
9%
|
Canada |
468,700
|
9%
|
Niger |
421,000
|
8%
|
South Africa |
279,100
|
5%
|
Brazil |
276,700
|
5%
|
Namibia |
261,000
|
5%
|
USA |
207,400
|
4%
|
China |
166,100
|
3%
|
Ukraine |
119,600
|
2%
|
Uzbekistan |
96,200
|
2%
|
Mongolia |
55,700
|
1%
|
Jordan |
33,800
|
1%
|
other |
164,000
|
3%
|
World total |
5,327,200
|
Reasonably Assured Resources plus Inferred Resources, to US$ 130/kg U, 1/1/11, from OECD NEA & IAEA, Uranium 2011: Resources, Production and Demand ("Red Book"). The total to US$ 260/kg U is 7,096,600 tonnes U, and Namibia moves up ahead of Niger.

Current usage is about 68,000 tU/yr. Thus the world's present
measured resources of uranium (5.3 Mt) in the cost category around
present spot prices and used only in conventional reactors, are enough
to last for about 80 years. This represents a higher level of assured
resources than is normal for most minerals. Further exploration and
higher prices will certainly, on the basis of present geological
knowledge, yield further resources as present ones are used up.
An initial uranium exploration cycle was military-driven, over 1945
to 1958. The second cycle was about 1974 to 1983, driven by civil
nuclear power and in the context of a perception that uranium might be
scarce. There was relatively little uranium exploration between 1985 and
2003, so the significant increase in exploration effort since then
could conceivably double the known economic resources despite
adjustments due to increasing costs. In the two years 2005-06 the
world’s known uranium resources tabulated above and graphed below
increased by 15% (17% in the cost category to $80/kgU). World uranium
exploration expenditure is increasing, as the the accompanying graph
makes clear. In the third uranium exploration cycle from 2003 to the end
of 2011 about US$ 10 billion was spent on uranium exploration and
deposit delineation on over 600 projects. In this period over 400 new
junior companies were formed or changed their orientation to raise over
US$ 2 billion for uranium exploration. About 60% of this was spent on
previously-known deposits. All this was in response to increased uranium
price in the market and the prospect of firm future prices.
The price of a mineral commodity also directly determines the amount
of known resources which are economically extractable. On the basis of
analogies with other metal minerals, a doubling of price from present
levels could be expected to create about a tenfold increase in measured
economic resources, over time, due both to increased exploration and the
reclassification of resources regarding what is economically
recoverable.
This is in fact suggested in the IAEA-NEA figures if those covering
estimates of all conventional resources (U as main product or major
by-product) are considered - another 7.6 million tonnes (beyond the 5.3
Mt known economic resources), which takes us to 190 years' supply at
today's rate of consumption. This still ignores the technological factor
mentioned below. It also omits unconventional resources (U recoverable
as minor by-product) such as phosphate/ phosphorite deposits (up to 22
Mt U), black shales (schists) and lignite (0.7 Mt U), and even seawater
(up to 4000 Mt), which would be uneconomic to extract in the foreseeable
future, although Japanese trials using a polymer braid have suggested
costs a bit over $250/kgU.
Research proceeds.
It is clear from this Figure that known uranium resources have
increased almost threefold since 1975, in line with expenditure on
uranium exploration. (The decrease in the decade 1983-93 is due to some
countries tightening their criteria for reporting. If this were carried
back two decades, the lines would fit even more closely. The change
from 2007 to 2009 is due to reclassifying resources into higher-cost
categories.) Increased exploration expenditure in the future is likely
to result in a corresponding increase in known resources, even as
inflation increases costs of recovery and hence tends to decrease the
figures in each cost category.
About 20% of US uranium came from central Florida's phosphate
deposits to the mid 1990s, as a by-product, but it then became
uneconomic. With higher uranium prices today the resource is being
examined again, as is another lower-grade one in Morocco. Plans for
Florida extend only to 400 tU/yr at this stage. See also companion paper
on Uranium from Phosphate Deposits.
Coal ash is another easily-accessible though minor uranium resource
in many parts of the world. In central Yunnan province in China the
coal uranium content varies up to 315 ppm and averages about 65 ppm.
The ash averages about 210 ppm U (0.021%U) - above the cut-off level for
some uranium mines. The Xiaolongtang power station ash heap contains
over 1000 tU, with annual arisings of 190 tU. Recovery of this by acid
leaching is about 70% in trials. This project has yet to announce any
commercial production, however.
Widespread use of the fast breeder reactor could increase the
utilisation of uranium 50-fold or more. This type of reactor can be
started up on plutonium derived from conventional reactors and operated
in closed circuit with its reprocessing plant. Such a reactor, supplied
with natural or depleted uranium for its "fertile blanket", can be
operated so that each tonne of ore yields 60 times more energy than in a
conventional reactor.
see also WNA position paper.
Reactor Fuel Requirements
The world’s power reactors, with combined capacity of some 375 GWe,
require about 68,000 tonnes of uranium from mines or elsewhere each
year. While this capacity is being run more productively, with higher
capacity factors and reactor power levels, the uranium fuel requirement
is increasing, but not necessarily at the same rate. The factors
increasing fuel demand are offset by a trend for higher burn-up of fuel
and other efficiencies, so demand is steady. (Over the years 1980 to
2008 the electricity generated by nuclear power increased 3.6-fold while
uranium used increased by a factor of only 2.5.)
Reducing the tails assay in enrichment reduces the amount of natural
uranium required for a given amount of fuel. Reprocessing of used fuel
from conventional light water reactors also utilises present resources
more efficiently, by a factor of about 1.3 overall.
Today's reactor fuel requirements are met from primary supply (direct
mine output - 78% in 2009) and secondary sources: commercial
stockpiles, nuclear weapons stockpiles, recycled plutonium and uranium
from reprocessing used fuel, and some from re-enrichment of depleted
uranium tails (left over from original enrichment). These various
secondary sources make uranium unique among energy minerals.
Nuclear Weapons as a source of fuel
An important source of nuclear fuel is the world's nuclear weapons
stockpiles. Since 1987 the United States and countries of the former
USSR have signed a series of disarmament treaties to reduce the nuclear
arsenals of the signatory countries by approximately 80 percent.
The weapons contained a great deal of uranium enriched to over 90
percent U-235 (ie up to 25 times the proportion in reactor fuel). Some
weapons have plutonium-239, which can be used in mixed-oxide (MOX) fuel
for civil reactors. From 2000 the dilution of 30 tonnes of military
high-enriched uranium has been displacing about 10,600 tonnes of uranium
oxide per year from mines, which represents about 15% of the world's
reactor requirements.
Details of the utilisation of military stockpiles are in the paper Military warheads as a source of nuclear fuel.
Other secondary sources of uranium
The most obvious source is civil stockpiles held by
utilities and governments. The amount held here is difficult to
quantify, due to commercial confidentiality. As at January 2009 some
129,000 tU total inventory was estimated for utilities, 10,000 tU for
producers and 15,000 tU for fuel cycle participants, making a total of
154,000 tU (WNA Market Report). These reserves are expected not to be
drawn down, but to increase steadily to provide energy security for
utilities and governments.
Recycled uranium and plutonium is another source,
and currently saves 1500-2000 tU per year of primary supply, depending
on whether just the plutonium or also the uranium is considered. In
fact, plutonium is quickly recycled as MOX fuel, whereas the reprocessed
uranium (RepU) is mostly stockpiled. See also Processing of Used Nuclear Fuel for Recycle paper.
Re-enrichment of depleted uranium (DU, enrichment
tails) is another secondary source. There is about 1.5 million tonnes
of depleted uranium available, from both military and civil enrichment
activity since the 1940s, most at tails assay of 0.25 - 0.35% U-235.
Non-nuclear uses of DU are very minor relative to annual arisings of
over 35,000 tU per year. This leaves most DU available for mixing with
recycled plutonium on MOX fuel or as a future fuel resource for fast
neutron reactors. However, some that has relatively high assay can be
fed through under-utilised enrichment plants to produce natural uranium
equivalent, or even enriched uranium ready for fuel fabrication.
Russian enrichment plants have treated 10-15,000 tonnes per year of DU
assaying over 0.3% U-235, stripping it down to 0.1% and producing a few
thousand tonnes per year of natural uranium equivalent. This Russian
program treating Western tails has now finished, but a new US one is
expected to start when surplus capacity is available, treating about
140,000 tonnes of old DU assaying 0.4% U-235.
International fuel reserves
There have been three major initiatives to set up international
reserves of enriched fuel, two of them multilateral ones, with fuel to
be available under International Atomic Energy Agency (IAEA) auspices
despite any political interruptions which might affect countries needing
them. The third is under US auspices, and also to meet needs arising
from supply disruptions.
In November 2009 the IAEA Board approved a Russian proposal to create an international "fuel bank" or guaranteed reserve of low-enriched uranium under IAEA control at the International Uranium Enrichment Centre (IUEC) at Angarsk. This Russian LEU reserve was established a year later and comprises 120 tonnes of low-enriched uranium as UF6, enriched 2.0 - 4.95% U-235 (with 40t of latter), available to any IAEA member state in good standing which is unable to procure fuel for political reasons. It is fully funded by Russia, held under safeguards, and the fuel will be made available to IAEA at market rates, using a formula based on spot prices. Following an IAEA decision to allocate some of it, Rosatom will transport material to St Petersburg and transfer title to IAEA, which will then transfer ownership to the recipient.
This initiative complements the proposed IAEA fuel bank by making more material available to the IAEA for assurance of fuel supply to countries without their own fuel cycle facilities. The 120 tonnes uranium as UF6 is equivalent to two full fuel loads for a typical 1000 MWe reactor, and is (in 2011) worth some US$ 250 million.
In December 2010 the IAEA board resolved to establish a similar guaranteed reserve of low-enriched uranium, the IAEA LEU bank, with the support of $50 million from the US-based Nuclear Threat Initiative (NTI) organization and US billionaire Warren Buffett, plus a matching $107 million from the US government ($50 million), the EU ($32 million), UAE ($10 million), Kuwait ($10 million) and Norway ($5 million). The IAEA is drawing up a framework that defines the "fuel bank's" structure, access and location. It will comprise a physical stock of UF6 at enrichment levels ranging up to 4.95% U-235 and owned by the IAEA, which shall "be responsible for storing and protecting" it. A comprehensive Host State Agreement will need to provide for the IAEA facility to be extraterritorial. Kazakhstan has offered to host it, and the IAEA has examined two proffered sites. See IAEA Factsheet.
In November 2009 the IAEA Board approved a Russian proposal to create an international "fuel bank" or guaranteed reserve of low-enriched uranium under IAEA control at the International Uranium Enrichment Centre (IUEC) at Angarsk. This Russian LEU reserve was established a year later and comprises 120 tonnes of low-enriched uranium as UF6, enriched 2.0 - 4.95% U-235 (with 40t of latter), available to any IAEA member state in good standing which is unable to procure fuel for political reasons. It is fully funded by Russia, held under safeguards, and the fuel will be made available to IAEA at market rates, using a formula based on spot prices. Following an IAEA decision to allocate some of it, Rosatom will transport material to St Petersburg and transfer title to IAEA, which will then transfer ownership to the recipient.
This initiative complements the proposed IAEA fuel bank by making more material available to the IAEA for assurance of fuel supply to countries without their own fuel cycle facilities. The 120 tonnes uranium as UF6 is equivalent to two full fuel loads for a typical 1000 MWe reactor, and is (in 2011) worth some US$ 250 million.
In December 2010 the IAEA board resolved to establish a similar guaranteed reserve of low-enriched uranium, the IAEA LEU bank, with the support of $50 million from the US-based Nuclear Threat Initiative (NTI) organization and US billionaire Warren Buffett, plus a matching $107 million from the US government ($50 million), the EU ($32 million), UAE ($10 million), Kuwait ($10 million) and Norway ($5 million). The IAEA is drawing up a framework that defines the "fuel bank's" structure, access and location. It will comprise a physical stock of UF6 at enrichment levels ranging up to 4.95% U-235 and owned by the IAEA, which shall "be responsible for storing and protecting" it. A comprehensive Host State Agreement will need to provide for the IAEA facility to be extraterritorial. Kazakhstan has offered to host it, and the IAEA has examined two proffered sites. See IAEA Factsheet.
In 2005 the US government announced plans for the establishment of a
mechanism to ensure fuel supply for use in commercial reactors in
foreign countries where there has been supply disruption. The fuel would
come from downblending 17.4 tonnes of high-enriched uranium (HEU). In
August 2011 US Department of Energy announced an expanded scope for the
program so it would also serve US utility needs, and now be called the American Assured Fuel Supply (AFS).
At that point most of the downblending of the HEU had been completed,
and the scheme was ready to operate. The AFS will comprise about 230
tonnes of low-enriched uranium (with another 60t from downblending being
sold on the market to pay for the work). The AFS program is
administered by the US National Nuclear Safety Administration, foreign
access must be through a US entity, and the fuel will be sold at current
market prices. The 230 t amount is equivalent to about six reloads for a
1000 MWe reactor.
Thorium as a nuclear fuel
Today uranium is the only fuel supplied for nuclear reactors.
However, thorium can also be utilised as a fuel for CANDU reactors or in
reactors specially designed for this purpose. Neutron efficient
reactors, such as CANDU, are capable of operating on a thorium fuel
cycle, once they are started using a fissile material such as U-235 or
Pu-239. Then the thorium (Th-232) atom captures a neutron in the reactor
to become fissile uranium (U-233), which continues the reaction. Some
advanced reactor designs are likely to be able to make use of thorium on
a substantial scale.
The thorium fuel cycle has some attractive features, though it is not
yet in commercial use. Thorium is reported to be about three times as
abundant in the earth's crust as uranium. The 2009 IAEA-NEA "Red Book"
lists 3.6 million tonnes of known and estimated resources as reported,
but points out that this excludes data from much of the world, and
estimates about 6 million tonnes overall. See also companion paper on Thorium.
Main references
OECD NEA & IAEA, 2010, Uranium 2009: Resources, Production and Demand
WNA 2009 Market Report
UN Institute for Disarmament Research, Yury Yudin (ed) 2011, Multilateralization of the Nuclear Fuel Cycle - The First Practical Steps.
OECD NEA & IAEA, 2010, Uranium 2009: Resources, Production and Demand
WNA 2009 Market Report
UN Institute for Disarmament Research, Yury Yudin (ed) 2011, Multilateralization of the Nuclear Fuel Cycle - The First Practical Steps.
Substantially derived from 2003 WNA Symposium paper by Colin MacDonald, Uranium: Sustainable Resource or Limit to Growth?
- supplemented by his 2005 WNA Symposium paper and including a model
"Economic adjustments in the supply of a 'non-renewable' resource" from
Ian Hore-Lacy.
The Sustainability of Mineral Resources
with reference to uranium
with reference to uranium
It is commonly asserted that because "the resources of the earth are
finite", therefore we must face some day of reckoning, and will need to
plan for "negative growth". All this, it is pointed out, is because
these resources are being consumed at an increasing rate to support our
western lifestyle and to cater for the increasing demands of developing
nations. The assertion that we are likely to run out of resources is a
re-run of the "Limits to Growth" argument (Club of Rome 1972 popularised
by Meadows et al in Limits of Growth at that time. (A useful counter to it is W Berckerman, In Defence of Economic Growth,
also Singer, M, Passage to a Human World, Hudson Inst. 1987). In the
decade following its publication world bauxite reserves increased 35%,
copper 25%, nickel 25%, uranium and coal doubled, gas increased 70% and
even oil increased 6%.) fashionable in the early 1970s, which was
substantially disowned by its originators, the Club of Rome, and shown
up as nonsense with the passing of time. It also echoes similar concerns
raised by economists in the 1930s, and by Malthus at the end of the
18th Century.
In recent years there has been persistent misunderstanding and
misrepresentation of the abundance of mineral resources, with the
assertion that the world is in danger of actually running out of many
mineral resources. While congenial to common sense if the scale of the
Earth's crust is ignored, it lacks empirical support in the trend of
practically all mineral commodity prices and published resource figures
over the long term. In recent years some have promoted the view that
limited supplies of natural uranium are the Achilles heel of nuclear
power as the sector contemplates a larger contribution to future clean
energy, notwithstanding the small amount of it required to provide very
large amounts of energy.
Uranium supply news is usually framed within a short-term
perspective. It concerns who is producing with what resources, who might
produce or sell, and how does this balance with demand? However,
long-term supply analysis enters the realm of resource economics. This
discipline has as a central concern the understanding of not just
supply/demand/price dynamics for known resources, but also the
mechanisms for replacing resources with new ones presently unknown. Such
a focus on sustainability of supply is unique to the long view.
Normally-functioning metals markets and technology change provide the
drivers to ensure that supply at costs affordable to consumers is
continuously replenished, both through the discovery of new resources
and the re-definition (in economic terms) of known ones.
Of course the resources of the earth are indeed finite, but three
observations need to be made: first, the limits of the supply of
resources are so far away that the truism has no practical meaning.
Second, many of the resources concerned are either renewable or
recyclable (energy minerals and zinc are the main exceptions, though the
recycling potential of many materials is limited in practice by the
energy and other costs involved). Third, available reserves of
'non-renewable' resources are constantly being renewed, mostly faster
than they are used.
There are three principal areas where resource predictions have faltered:
- predictions have not accounted for gains in geological knowledge and understanding of mineral deposits;
- they have not accounted for technologies utilised to discover, process and use them;
- economic principles have not been taken into account, which means that resources are thought of only in present terms, not in terms of what will be economic through time, nor with concepts of substitution in mind.
What then does sustainability in relation to mineral resources mean?
The answer lies in the interaction of these three things which enable
usable resources (Some licence is taken in the use of this word in the
following, strictly it is reserves of minerals which are created)
effectively to be created. They are brought together in the diagram
below.

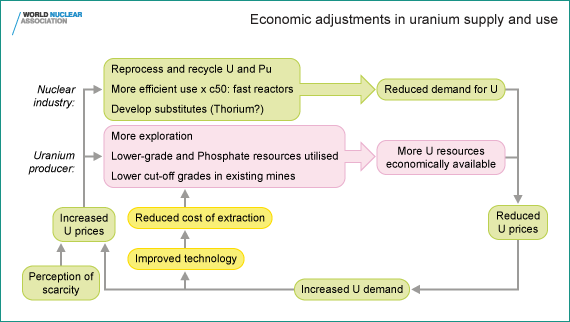
Numerous economists have studied resource trends to determine which measures should best reflect resource scarcity (Tilton, J. On Borrowed Time? Assessing the threat of mineral depletion,
Resources for the Future, Washington DC 2002). Their consensus view is
that costs and prices, properly adjusted for inflation, provide a better
early warning system for long-run resource scarcity than do physical
measures such as resource quantities.
Historic data show that the most commonly used metals have declined
in both their costs and real commodity prices over the past century.
Such price trends are the most telling evidence of lack of scarcity.
Uranium has been a case in point, relative to its late 1970s price of
US$ 40/lb U3O8.
An anecdote underlines this basic truth: In 1980 two eminent
professors, fierce critics of one another, made a bet regarding the real
market price of five metal commodities over the next decade. Paul
Ehrlich, a world-famous ecologist, bet that because the world was
exceeding its carrying capacity, food and commodities would start to run
out in the 1980s and prices in real terms would therefore rise. Julian
Simon, an economist, said that resources were effectively so abundant,
and becoming effectively more so, that prices would fall in real terms.
He invited Ehrlich to nominate which commodities would be used to test
the matter, and they settled on these (chrome, copper, nickel, tin and
tungsten). In 1990 Ehrlich paid up - all the prices had fallen.
However, quantities of known resources tell a similar and consistent
story. To cite one example, world copper reserves in the 1970s
represented only 30 years of then-current production (6.4 Mt/yr). Many
analysts questioned whether this resource base could satisfy the large
expected requirements of the telecommunications industry by 2000. But by
1994, world production of copper had doubled (12 Mt/yr) and the
available reserves were still enough for another 30 years. The reserve
multiple of current production remained the same.
Metal Prices
Another way to understand resource sustainability is in terms of
economics and capital conservation. Under this perspective, mineral
resources are not so much rare or scarce as they are simply too
expensive to discover if you cannot realise the profits from your
discovery fairly soon. Simple economic considerations therefore
discourage companies from discovering much more than society needs
through messages of reduced commodity prices during times of oversupply.
Economically rational players will only invest in finding these new
reserves when they are most confident of gaining a return from them,
which usually requires positive price messages caused by undersupply
trends. If the economic system is working correctly and maximizing
capital efficiency, there should never be more than a few decades of any
resource commodity in reserves at any point in time.
Resource levels
The fact that many commodities have more resources available than
efficient economic theory might suggest may be partly explained by two
characteristics of mineral exploration cycles. First, the exploration
sector tends to over-respond to the positive price signals through rapid
increases in worldwide expenditures (which increases the rate of
discoveries), in particular through the important role of more
speculatively-funded junior exploration companies. Exploration also
tends to make discoveries in clusters that have more to do with new
geological knowledge than with efficient capital allocation theory. As
an example, once diamonds were known to exist in northern Canada, the
small exploration boom that accompanied this resulted in several large
discoveries - more than the market may have demanded at this time. These
patterns are part of the dynamics that lead to commodity price cycles.
New resource discoveries are very difficult to precisely match with
far-off future demand, and the historic evidence suggests that the
exploration process over-compensates for every small hint of scarcity
that the markets provide.
Another important element in resource economics is the possibility of
substitution of commodities. Many commodity uses are not exclusive -
should they become too expensive they can be substituted with other
materials. Even if they become cheaper they may be replaced, as
technology gains have the potential to change the style and cost of
material usage. For example, copper, despite being less expensive in
real terms than 30 years ago, is still being replaced by fibre optics in
many communication applications. These changes to materials usage and
commodity demand provide yet another dimension to the simple notion of
depleting resources and higher prices.
In summary, historic metals price trends, when examined in the light
of social and economic change through time, demonstrate that resource
scarcity is a double-edged sword. The same societal trends that have
increased metals consumption, tending to increase prices, have also
increased the available wealth to invest in price-reducing knowledge and
technology. These insights provide the basis for the economic
sustainability of metals, including uranium.
Geological Knowledge
Whatever minerals are in the earth, they cannot be considered usable
resources unless they are known. There must be a constant input of time,
money and effort to find out what is there. This mineral exploration
endeavour is not merely fossicking or doing aerial magnetic surveys, but
must eventually extend to comprehensive investigation of orebodies so
that they can reliably be defined in terms of location, quantity and
grade. Finally, they must be technically and economically quantified as
mineral reserves. That is the first aspect of creating a resource. See
Appendix 2 for mineral resource and reserve categories.
For reasons outlined above, measured resources of many minerals are
increasing much faster than they are being used, due to exploration
expenditure by mining companies and their investment in research. Simply
on geological grounds, there is no reason to suppose that this trend
will not continue. Today, proven mineral resources worldwide are more
than we inherited in the 1970s, and this is especially so for uranium.
Simply put, metals which are more abundant in the Earth's crust are
more likely to occur as the economic concentrations we call mineral
deposits. They also need to be reasonably extractable from their host
minerals. By these measures, uranium compares very well with base and
precious metals. Its average crustal abundance of 2.7 ppm is comparable
with that of many other metals such as tin, tungsten, and molybdenum.
Many common rocks such as granite and shales contain even higher uranium
concentrations of 5 to 25 ppm. Also, uranium is predominantly bound in
minerals which are not difficult to break down in processing.
As with crustal abundance, metals which occur in many different kinds
of deposits are easier to replenish economically, since exploration
discoveries are not constrained to only a few geological settings.
Currently, at least 14 different types of uranium deposits are known,
occurring in rocks of wide range of geological age and geographic
distribution. There are several fundamental geological reasons why
uranium deposits are not rare, but the principal reason is that uranium
is relatively easy both to place into solution over geological time, and
to precipitate out of solution in chemically reducing conditions. This
chemical characteristic alone allows many geological settings to provide
the required hosting conditions for uranium resources. Related to this
diversity of settings is another supply advantage ?the wide range in the
geological ages of host rocks ensures that many geopolitical regions
are likely to host uranium resources of some quality.
Unlike the metals which have been in demand for centuries, society
has barely begun to utilise uranium. As serious non-military demand did
not materialise until significant nuclear generation was built by the
late 1970s, there has been only one cycle of
exploration-discovery-production, driven in large part by late 1970s
price peaks (MacDonald, C, Rocks to reactors: Uranium exploration and the market.
Proceedings of WNA Symposium 2001). This initial cycle has provided
more than enough uranium for the last three decades and several more to
come. Clearly, it is premature to speak about long-term uranium scarcity
when the entire nuclear industry is so young that only one cycle of
resource replenishment has been required. It is instead a reassurance
that this first cycle of exploration was capable of meeting the needs of
more than half a century of nuclear energy demand.
Related to the youthfulness of nuclear energy demand is the early
stage that global exploration had reached before declining uranium
prices stifled exploration in the mid 1980s. The significant investment
in uranium exploration during the 1970-82 exploration cycle would have
been fairly efficient in discovering exposed uranium deposits, due to
the ease of detecting radioactivity. Still, very few prospective regions
in the world have seen the kind of intensive knowledge and
technology-driven exploration that the Athabasca Basin of Canada has
seen since 1975. This fact has huge positive implications for future
uranium discoveries, because the Athabasca Basin history suggests that
the largest proportion of future resources will be as deposits
discovered in the more advanced phases of exploration. Specifically,
only 25% of the 635,000 tonnes of U3O8 discovered
so far in the Athabasca Basin could be discovered during the first phase
of surface-based exploration. A sustained second phase, based on
advances in deep penetrating geophysics and geological models, was
required to discover the remaining 75%.
Another dimension to the immaturity of uranium exploration is that it
is by no means certain that all possible deposit types have even been
identified. Any estimate of world uranium potential made only 30 years
ago would have missed the entire deposit class of unconformity deposits
that have driven production since then, simply because geologists did
not know this class existed.
Technology
It is meaningless to speak of a resource until someone has thought of
a way to use any particular material. In this sense, human ingenuity
quite literally creates new resources, historically, currently and
prospectively. That is the most fundamental level at which technology
creates resources, by making particular minerals usable in new ways.
Often these then substitute to some degree for others which are becoming
scarcer, as indicated by rising prices. Uranium was not a resource in
any meaningful sense before 1940.
More particularly, if a known mineral deposit cannot be mined,
processed and marketed economically, it does not constitute a resource
in any practical sense. Many factors determine whether a particular
mineral deposit can be considered a usable resource - the scale of
mining and processing, the technological expertise involved, its
location in relation to markets, and so on. The application of human
ingenuity, through technology, alters the significance of all these
factors and is thus a second means of "creating" resources. In effect,
portions of the earth's crust are reclassified as resources. A further
aspect of this is at the manufacturing and consumer level, where
technology can make a given amount of resources go further through more
efficient use.(aluminium can mass was reduced by 21% 1972-88, and motor
cars each use about 30% less steel than 30 years ago)
An excellent example of this application of technology to create
resources is in the Pilbara region of Western Australia. Until the 1960s
the vast iron ore deposits there were simply geological curiosities,
despite their very high grade. Australia had been perceived as short of
iron ore. With modern large-scale mining technology and the advent of
heavy duty railways and bulk shipping which could economically get the
iron ore from the mine (well inland) through the ports of Dampier and
Port Hedland to Japan, these became one of the nation's main mineral
resources. For the last 45 years Hamersley Iron (Rio Tinto), Mount
Newman (BHP-Billiton) and others have been at the forefront of
Australia's mineral exporters, drawing upon these 'new' orebodies.
Just over a hundred years ago aluminium was a precious metal, not
because it was scarce, but because it was almost impossible to reduce
the oxide to the metal, which was therefore fantastically expensive.
With the discovery of the Hall-Heroult process in 1886, the cost of
producing aluminium plummeted to about one twentieth of what it had been
and that metal has steadily become more commonplace. It now competes
with iron in many applications, and copper in others, as well as having
its own widespread uses in every aspect of our lives. Not only was a
virtually new material provided for people's use by this technological
breakthrough, but enormous quantities of bauxite world-wide
progressively became a valuable resource. Without the technological
breakthrough, they would have remained a geological curiosity.
Incremental improvements in processing technology at all plants are
less obvious but nevertheless very significant also. Over many years
they are probably as important as the historic technological
breakthroughs.
To achieve sustainability, the combined effects of mineral
exploration and the development of technology need to be creating
resources at least as fast as they are being used. There is no question
that in respect to the minerals industry this is generally so, and with
uranium it is also demonstrable. Recycling also helps, though generally
its effect is not great.
Economics
Whether a particular mineral deposit is sensibly available as a
resource will depend on the market price of the mineral concerned. If it
costs more to get it out of the ground than its value warrants, it can
hardly be classified as a resource (unless there is some major market
distortion due to government subsidies of some kind). Therefore, the
resources available will depend on the market price, which in turn
depends on world demand for the particular mineral and the costs of
supplying that demand. The dynamic equilibrium between supply and demand
also gives rise to substitution of other materials when scarcity looms
(or the price is artificially elevated). This then is the third aspect
of creating resources.
The best known example of the interaction of markets with resource
availability is in the oil industry. When in 1972 OPEC suddenly
increased the price of oil fourfold, several things happened at both
producer and consumer levels.
The producers dramatically increased their exploration effort, and
applied ways to boost oil recovery from previously 'exhausted' or
uneconomic wells. At the consumer end, increased prices meant massive
substitution of other fuels and greatly increased capital expenditure in
more efficient plant. As a result of the former activities, oil
resources increased dramatically. As a result of the latter, oil use
fell slightly to 1975 and in the longer perspective did not increase
globally from 1973 to 1986. Forecasts in 1972, which had generally
predicted a doubling of oil consumption in ten years, proved quite
wrong.
Oil will certainly become scarce one day, probably before most other
mineral resources, which will continue to drive its price up. As in the
1970s, this will in turn cause increased substitution for oil and bring
about greater efficiencies in its use as equilibrium between supply and
demand is maintained by the market mechanism. Certainly oil will never
run out in any absolute sense - it will simply become too expensive to
use as liberally as we now do.
Another example is provided by aluminium. During World War II,
Germany and Japan recovered aluminium from kaolinite, a common clay, at
slightly greater cost than it could be obtained from bauxite.
Due to the operation of these three factors the world's economically
demonstrated resources of most minerals have risen faster than the
increased rate of usage over the last 50 years, so that more are
available now, notwithstanding liberal usage. This is largely due to the
effects of mineral exploration and the fact that new discoveries have
exceeded consumption.
Replacement of uranium
A characteristic of metals resource replacement is that the mineral
discovery process itself adds a small cost relative to the value of the
discovered metals. As an example, the huge uranium reserves of Canada's
Athabasca Basin were discovered for about US$1.00/kgU (2003 dollars,
including unsuccessful exploration). Similar estimates for world uranium
resources, based on published IAEA exploration expenditure data and
assuming that these expenditures yielded only the past uranium produced
plus the present known economic resources categories at up to US$80/kg (Uranium 2003: Resources, Production and demand.
Nuclear Energy Agency and IAEA, OECD Publications 2004) yields slightly
higher costs of about US$1.50/kgU. This may reflect the higher
component of State-driven exploration globally, some of which had
national self-sufficiency objectives that may not have aligned with
industry economic standards.
From an economic perspective, these exploration costs are essentially
equivalent to capital investment costs, albeit spread over a longer
time period. It is, however, this time lag between the exploration
expense and the start of production that confounds attempts to analyse
exploration economics using strict discounted cash flow methods. The
positive cash flows from production occur at least 10-15 years into the
future, so that their present values are obviously greatly reduced,
especially if one treats the present as the start of exploration. This
creates a paradox, since large resource companies must place a real
value on simply surviving and being profitable for many decades into the
future; and, without exploration discoveries, all mining companies must
expire with their reserves. Recent advances in the use of real options
and similar methods are providing new ways to understand this apparent
paradox. A key insight is that time, rather than destroying value
through discounting, actually adds to the option value, as does the
potential of price volatility. Under this perspective, resource
companies create value by obtaining future resources which can be
exploited optimally under a range of possible economic conditions.
Techniques such as these are beginning to add analytical support to what
have always been intuitive understandings by resource company leaders -
that successful exploration creates profitable mines and adds value to
company shares.
Since uranium is part of the energy sector, another way to look at
exploration costs is on the basis of energy value. This allows
comparisons with the energy investment cost for other energy fuels,
especially fossil fuels which will have analogous costs related to the
discovery of the resources. From numerous published sources, the finding
costs of crude oil have averaged around US$ 6/bbl over at least the
past three decades. When finding costs of the two fuels are expressed in
terms of their contained energy value, oil, at US$ 1050/MJ of energy,
is about 300 times more expensive to find than uranium, at US$ 3.4/MJ.
Similarly, the proportion of current market prices that finding costs
comprise are lower for uranium. Its finding costs make up only 2% of the
recent spot price of US$ 30/lb ($78/kgU), while the oil finding costs
are 12% of a recent spot price of US$ 50/bbl.
By these measures, uranium is a very inexpensive energy source to
replenish, as society has accepted far higher energy replacement costs
to sustain oil resources. This low basic energy resource cost is one
argument in favour of a nuclear-hydrogen solution to long-term
replacement of oil as a transportation fuel.
Forecasting replenishment
Supply forecasters are often reluctant to consider the additive
impacts of exploration on new supply, arguing that assuming discoveries
is as risky and speculative as the exploration business itself. Trying
to predict any single discovery certainly is speculative. However, as
long as the goal is merely to account for the estimated total discovery
rate at a global level, a proxy such as estimated exploration
expenditures can be used. Since expenditures correlate with discovery
rate, the historic (or adjusted) resources discovered per unit of
expenditure will provide a reasonable estimate of resource gains to be
expected. As long as the time lag between discovery and production is
accounted for, this kind of dynamic forecasting is more likely to
provide a basis for both price increases and decreases, which metals
markets have historically demonstrated.
Without these estimates of uranium resource replenishment through
exploration cycles, long-term supply-demand analyses will tend to have a
built-in pessimistic bias (i.e. towards scarcity and higher prices),
that will not reflect reality. Not only will these forecasts tend to
overestimate the price required to meet long-term demand, but the
opponents of nuclear power use them to bolster arguments that nuclear
power is unsustainable even in the short term. In a similar fashion,
these finite-resources analyses also lead observers of the industry to
conclude that fast breeder reactor technology will soon be required.
This may indeed make a gradual appearance, but if uranium follows the
price trends we see in other metals, its development will be due to
strategic policy decisions more than uranium becoming too expensive.
The resource economics perspective tells us that new exploration
cycles should be expected to add uranium resources to the world
inventory, and to the extent that some of these may be of higher quality
and involve lower operating cost than resources previously identified,
this will tend to mitigate price increases. This is precisely what has
happened in uranium, as the low-cost discoveries in Canada's Athabasca
Basin have displaced higher-cost production from many other regions,
lowering the cost curve and contributing to lower prices. Secondary
uranium supplies, to the extent that they can be considered as a very
low-cost mine, have simply extended this price trend.
The first exploration and mining cycle for uranium occurred about
1970 to 1985. It provided enough uranium to meet world demand for some
80 years, if we view present known resources as arising from it. With
the rise in uranium prices to September 2005 and the concomitant
increase (boom?) in mineral exploration activity, it is clear that we
have the start of a second such cycle, mid 2003 to ??. The price
increase was brought about by diminution of secondary supplies coupled
with a realization that primary supplies needed to increase
substantially.
Several significant decisions on mine development and increased
exploration by major producers will enable this expansion of supply,
coupled with smaller producers coming on line. The plethora of junior
exploration companies at the other end of the spectrum which are finding
no difficulty whatever in raising capital are also a positive sign that
a vigorous new exploration and mining cycle is cranking up. From lows
of around US$ 55 million per year in 2000, world uranium exploration
expenditure rose to about US$ 110 million in 2004 and is expected to be
US$ 185 million in 2005, half of this being from the junior exploration
sector. The new cycle is also showing considerable regional
diversification. Measured from 1990, cycle 2 totals US$ 1.5 billion to
2005, compared with a total of about three times this figure
(uncorrected) for the whole of the first cycle.
Depletion and sustainability
Conversely, the exhaustion of mineral resources during mining is
real. Resource economists do not deny the fact of depletion, nor its
long-term impact - that in the absence of other factors, depletion will
tend to drive commodity prices up. But as we have seen, mineral
commodities can become more available or less scarce over time if the
cost-reducing effects of new technology and exploration are greater than
the cost-increasing effects of depletion.
One development that would appear to argue against economic
sustainability is the growing awareness of the global depletion of oil,
and in some regions such as North America, natural gas. But oil is a
fundamentally different material. This starts with geology, where key
differences include the fact that oil and gas were formed by only one
process: the breakdown of plant life on Earth. Compared with the immense
volumes of rock-forming minerals in the Earth? crust, living organisms
on top of it have always been a very tiny proportion. But a more
important fact is that the world has consumed oil, and recently natural
gas as well, in a trajectory of rapid growth virtually unmatched by any
other commodity. Consumption growth rates of up to 10% annually over the
past 50 years are much higher than we see for other commodities, and
support the contention that oil is a special depletion case for several
reasons: its geological occurrence is limited, it has been inexpensive
to extract, its energy utility has been impossible to duplicate for the
price, and its resulting depletion rates have been incredibly high.
This focus on rates of depletion suggests that one of the dimensions
of economic sustainability of metals has to do with their relative rates
of depletion. Specifically, it suggests that economic sustainability
will hold indefinitely as long as the rate of depletion of mineral
resources is slower than the rate at which it is offset. This offsetting
force will be the sum of individual factors that work against
depletion, and include cost-reducing technology and knowledge, lower
cost resources through exploration advances, and demand shifting through
substitution of materials.
An economic sustainability balance of this type also contemplates
that, at some future point, the offsetting factors may not be sufficient
to prevent irreversible depletion-induced price increases, and it is at
this point that substituting materials and technologies must come into
play to take away demand. In the case of rapid oil depletion, that
substitute appears to be hydrogen as a transport fuel. Which raises the
question of how the hydrogen is produced, and nuclear energy seems the
most likely means of that, using high-temperature reactors.
From a detached viewpoint all this may look like mere technological
optimism. But to anyone closely involved it is obvious and demonstrable.
Furthermore, it is illustrated by the longer history of human use of
the Earth's mineral resources. Abundance, scarcity, substitution,
increasing efficiency of use, technological breakthroughs in discovery,
recovery and use, sustained incremental improvements in mineral recovery
and energy efficiency - all these comprise the history of minerals and
humankind.
Appendix 2.
Mineral Resources and Reserves
The International Template for Reporting of Exploration
Results, Mineral Resources and Mineral Reserves (July 2006) integrates
the minimum standards being adopted in national reporting codes
worldwide with recommendations and interpretive guidelines for the
Public Reporting of Exploration Results, Mineral Resources and Mineral
Reserves. The definitions (below) in this edition of the International
Reporting Template are either identical to, or not materially different
from those definitions used in the countries represented on the
Committee for Mineral Reserves International Reporting Standards
(CRIRSCO), notably Australia, whose JORC code was the basis of these
international definitions, and Canada (NI 43-101 code).
A ‘Mineral Resource’ is a concentration or occurrence of material of intrinsic economic interest in or on the Earth’s crust in such form, quality and quantity that here are reasonable prospects for eventual economic extraction. The location, quantity, grade, geological characteristics and continuity of a Mineral Resource are known, estimated or interpreted from specific geological evidence, sampling and knowledge. Mineral Resources are sub-divided, in order of increasing geological confidence, into Inferred, Indicated and Measured categories.
An ‘Inferred Mineral Resource’ is that part of a Mineral Resource for which tonnage, grade and mineral content can be estimated with a low level of confidence. It is inferred from geological evidence and assumed but not verified geological and/or grade continuity. It is based on information gathered through appropriate techniques from locations such as outcrops, trenches, pits, workings and drill holes which is limited or of uncertain quality and reliability.
An ‘Indicated Mineral Resource’ is that part of a Mineral Resource for which tonnage, densities, shape, physical characteristics, grade and mineral content can be estimated with a reasonable level of confidence. It is based on exploration, sampling and testing information gathered through appropriate techniques from locations such as outcrops, trenches, pits, workings and drill holes. The locations are too widely or inappropriately spaced to confirm geological and/or grade continuity but are spaced closely enough for continuity to be assumed.
A ‘Measured Mineral Resource’ is that part of a Mineral Resource for which tonnage, densities, shape, physical characteristics, grade and mineral content can be estimated with a high level of confidence. It is based on detailed and reliable exploration, sampling and testing information gathered through appropriate techniques from locations such as outcrops, trenches, pits, workings and drill holes. The locations are spaced closely enough to confirm geological and grade continuity.
A ‘Mineral Reserve’ (or Ore Reserve) is the economically mineable part of a Measured and/or Indicated Mineral Resource. It includes diluting materials and allowances for losses, which may occur when the material is mined. Appropriate assessments and studies will have been carried out, and include consideration of and modification by realistically assumed mining, metallurgical, economic, marketing, legal, environmental, social and governmental factors. These assessments demonstrate at the time of reporting that extraction could reasonably be justified. Mineral or Ore Reserves are sub-divided in order of increasing confidence into Probable Mineral/Ore Reserves and Proved Mineral/Ore Reserves.
A ‘Probable Mineral Reserve’ (or Probable Ore Reserve) is the economically mineable part of an Indicated, and in some circumstances, a Measured Mineral Resource. It includes diluting materials and allowances for losses which may occur when the material is mined. Studies to at least Pre-Feasibility level will have been carried out, including consideration of and modification by realistically assumed mining, metallurgical, economic, marketing, legal, environmental, social and governmental factors. The results of the studies demonstrate at the time of reporting that extraction could reasonably be justified.
A ‘Proved Mineral Reserve’ (or proved Ore Reserve) is the economically mineable part of a Measured Mineral Resource. It includes diluting materials and allowances for losses which may occur when the material is mined. Studies to at least Pre-Feasibility level will have been carried out, including consideration of and modification by realistically assumed mining, metallurgical, economic, marketing, legal, environmental, social and governmental factors. These studies demonstrate at the time of reporting that extraction is justified.
About 20% of US uranium came from central Florida's phosphate deposits to the mid 1990s, as a by-product, but it then became uneconomic. From 1981 to 1992 US production averaged just over 1000 tU per year, then fell way sharply and finished in 1998. The IAEA "Red Book" also reports significant US production 1954-62. With higher uranium prices today the US resource is being examined again, as is a lower-grade one in Morocco. Plans for Florida extend only to 400 tU/yr at this stage.
Cameco and Uranium Equities Ltd are setting up a demonstration plant in the USA using a refined process – PhosEnergy – and estimate that some 7700 tU could be recovered annually as by-product from phosphate production, including 2300 tU/yr in the USA.
Phosphate rock (phosphorite) is a marine sedimentary rock which contains 18-40% P2O5, as well as some uranium and all its decay products, often 70 to 200 ppmU, and sometimes up to 800 ppm. The main mineral in the phosphate rock is apatite, and most commonly, fluorapatite – Ca5(PO4)3F or Ca10(PO4)6(F,OH)2. This is insoluble, so cannot directly be used as a fertiliser (unless in very acid soils) so must first be processed. This is normally in a wet process phosphoric acid (WPA) plant where it is first dissolved in sulphuric acid. About 2-4% fluorine is usually present. There are about 400 plants using this wet process worldwide, with capacity of some 50 million tonnes P2O5 per year.
Some phosphate deposits – about 4% of total known – are igneous, created by magmatic extrusion as an alkaline chimney. The main mineral is apatite, with some fluorapatite.
When phosphate rock is treated with sulphuric acid in sub stoichiometric quantity, normal superphosphate is formed. If more sulphuric acid is added, a mixture of phosphoric acid and gypsum (calcium sulphate) is obtained. After the gypsum is filtered out, the resultant phosphoric acid can be treated to recover uranium.
The basic reaction is:
Ca3(PO4)2 + 3H2SO4 + 6H2O ==> 2H3PO4 + 3CaSO4.2H2O – exothermic
An improved higher-temperature process produces hemihydrate: CaSO4.1/2H2O
Fluorides need to be controlled as gases and in effluents (HF, fluorosilicic acid) and about half the fluorine reports with the gypsum. In the process a lot of crud is generated, and this was disposed of with gypsum tailings, despite its low-level radioactivity.
After the gypsum precipitation stage, triple superphosphate is obtained by reacting the phosphoric acid with further phosphate rock. Otherwise, various ammonium phosphate fertilizers can be produced by reacting the phosphoric acid with ammonia.
The uranium is normally recovered from the phosphoric acid (H3PO4 – bearing about 28% P2O5) by some form of solvent extraction (SX). Kamorphos is developing a simpler version of this.
PhosEnergy, an ion exchange (IX) process, represents a step-change refinement of the old processes. It was announced in 2009, offering uranium recovery costs of $25-30 /lb U3O8, compared with historical costs of double this.a A demonstration plant was built in Adelaide, South Australia, and shipped to the USA to operate at a US fertilizer producer, where it was commissioned in May 2012. The test campaign with four trials on two feed sources was successful, showing recoveries of over 92% at $20-25/lb operating cost, and with Cameco very fully involved. Full evaluation of the project operation with an engineering study was reported in March 2013, and $18/lb operating cost with capital cost of $156 million for a base case 400 t/yr U3O8 plant were quoted. Cameco reaffirmed its commitment in subscribing a further $4 million.
The process involves taking 27% acid, redox reduction to ferrous iron giving oxidation of uranium, primary IX ,then recycle of acid with improved quality and a secondary IX uranium recovery being much the same as that in Cameco’s US ISL operations, so potential synergy. Bottom line of process is 95% U recovery, no radwaste, $18/lb cost, improved acid quality for main plant, $120 million bolt-on plant cost.
The next phase of PhosEnergy commercialisation is expected to be a continuous on-site demonstration scale operation at the site of an existing phosphate producer. This phase will underpin a Definitive Feasibility Study (DFS) and be the basis for a full-scale commercial facility, which might be constructed and commissioned within three years of the commencement of a DFS.
As well as the PhosEnergy project, Cameco is involved independently through Nukem with CF Industries in developing a facility to recover about 400 tU/yr from Florida phosphates.
In the USA eight plants for the recovery of uranium from phosphoric acid have been built and operated since the 1970s (six in Florida, two in Louisiana). Plants have also been built in Canada, Spain, Belgium (for Moroccan phosphate), Israel, and Taiwan. Brazil is planning a new plant with uranium as co-product with phosphate from 0.08%U ore in igneous rock, to operate from 2012 at 1000 tU/yr. Morocco has by far the largest known resources of uranium in phosphate rock. Egypt reports 42,000 tU in phosphate rocks, at 50-200 ppm U.
The potential amount of uranium able to be recovered from WPA phosphoric acid streams is over 11,000 tonnes U per year (global P2O5 production in 2010 was 33.6 Mt). The economic benefit will be both in the value of the uranium and in reduced regulatory demands on disposal of low-level radioactive wastes arising from the WPA process. Estimated uranium production costs will put the new process in the lowest quartile of new uranium production.
Santa Quiteria and Itataia mines, Brazil
This has reserves of 340 Mt of phosphate containing 140,000 tU at Santa Quiteria and 80,000 tU at Itataia, grading 0.054% U in P2O5, giving 1270 tU/yr from about 2015, 970 tU of this from Itataia.
USA
The country has reserves of 1400 Mt phosphates containing 170,000 tU. At 9.6 Mt/yr P2O5 production, this would yield 2300-2680 tU/yr by-product. Nukem and CF Industries were planning a 430 tU/yr uranium recovery operation at CF's Plant City.
Jordan
The country has reserves of 1500 Mt phosphates containing up to 140,000 tU. At 676,000 t/yr P2O5 production the uranium potential is 135 tU/yr. The government is putting out the Qatrana phosphorites for tender to develop, containing 52 Mt phosphate and 22,000 tU with vanadium.
Egypt
The country has reserves of 100 Mt phosphates containing 40,000 tU.
Tunisia
The country has reserves of 100 Mt phosphates containing 50,000 tU. At 1.6 Mt/yr P2O5 production, this would yield 265 tU/yr by-product.
Morocco
The country has reserves of 50 billion tonnes of phosphates containing 6.9 MtU. At 4.8 Mt/yr P2O5 production, this would yield 960 tU/yr by-product. There is some expectation of 1900 tU/yr production from 2017.
WISE, Uranium recovery from phosphates
A ‘Mineral Resource’ is a concentration or occurrence of material of intrinsic economic interest in or on the Earth’s crust in such form, quality and quantity that here are reasonable prospects for eventual economic extraction. The location, quantity, grade, geological characteristics and continuity of a Mineral Resource are known, estimated or interpreted from specific geological evidence, sampling and knowledge. Mineral Resources are sub-divided, in order of increasing geological confidence, into Inferred, Indicated and Measured categories.
An ‘Inferred Mineral Resource’ is that part of a Mineral Resource for which tonnage, grade and mineral content can be estimated with a low level of confidence. It is inferred from geological evidence and assumed but not verified geological and/or grade continuity. It is based on information gathered through appropriate techniques from locations such as outcrops, trenches, pits, workings and drill holes which is limited or of uncertain quality and reliability.
An ‘Indicated Mineral Resource’ is that part of a Mineral Resource for which tonnage, densities, shape, physical characteristics, grade and mineral content can be estimated with a reasonable level of confidence. It is based on exploration, sampling and testing information gathered through appropriate techniques from locations such as outcrops, trenches, pits, workings and drill holes. The locations are too widely or inappropriately spaced to confirm geological and/or grade continuity but are spaced closely enough for continuity to be assumed.
A ‘Measured Mineral Resource’ is that part of a Mineral Resource for which tonnage, densities, shape, physical characteristics, grade and mineral content can be estimated with a high level of confidence. It is based on detailed and reliable exploration, sampling and testing information gathered through appropriate techniques from locations such as outcrops, trenches, pits, workings and drill holes. The locations are spaced closely enough to confirm geological and grade continuity.
A ‘Mineral Reserve’ (or Ore Reserve) is the economically mineable part of a Measured and/or Indicated Mineral Resource. It includes diluting materials and allowances for losses, which may occur when the material is mined. Appropriate assessments and studies will have been carried out, and include consideration of and modification by realistically assumed mining, metallurgical, economic, marketing, legal, environmental, social and governmental factors. These assessments demonstrate at the time of reporting that extraction could reasonably be justified. Mineral or Ore Reserves are sub-divided in order of increasing confidence into Probable Mineral/Ore Reserves and Proved Mineral/Ore Reserves.
A ‘Probable Mineral Reserve’ (or Probable Ore Reserve) is the economically mineable part of an Indicated, and in some circumstances, a Measured Mineral Resource. It includes diluting materials and allowances for losses which may occur when the material is mined. Studies to at least Pre-Feasibility level will have been carried out, including consideration of and modification by realistically assumed mining, metallurgical, economic, marketing, legal, environmental, social and governmental factors. The results of the studies demonstrate at the time of reporting that extraction could reasonably be justified.
A ‘Proved Mineral Reserve’ (or proved Ore Reserve) is the economically mineable part of a Measured Mineral Resource. It includes diluting materials and allowances for losses which may occur when the material is mined. Studies to at least Pre-Feasibility level will have been carried out, including consideration of and modification by realistically assumed mining, metallurgical, economic, marketing, legal, environmental, social and governmental factors. These studies demonstrate at the time of reporting that extraction is justified.
Uranium from Phosphates
(Updated June 2013)- Rock phosphate deposits contain many million tonnes of uranium, which may be extracted as a by-product of making fertilisers.
- Some 20,000 tonnes of uranium has already been obtained from these rock phosphate deposits, but the process became uneconomic in the 1990s.
- Higher uranium prices and process refinements have changed the economic situation.
About 20% of US uranium came from central Florida's phosphate deposits to the mid 1990s, as a by-product, but it then became uneconomic. From 1981 to 1992 US production averaged just over 1000 tU per year, then fell way sharply and finished in 1998. The IAEA "Red Book" also reports significant US production 1954-62. With higher uranium prices today the US resource is being examined again, as is a lower-grade one in Morocco. Plans for Florida extend only to 400 tU/yr at this stage.
Cameco and Uranium Equities Ltd are setting up a demonstration plant in the USA using a refined process – PhosEnergy – and estimate that some 7700 tU could be recovered annually as by-product from phosphate production, including 2300 tU/yr in the USA.
Phosphate rock production for fertilizer in 2010, million tonnes
Algeria | 2 |
---|---|
Australia | 2.8 |
Brazil | 5.5 |
China | 65 |
Egypt | 5 |
Israel | 3 |
Jordan | 6 |
Morocco & W. Sahara | 26 |
Russia | 10 |
South Africa | 2.3 |
Syria | 2.8 |
Tunisia | 7.6 |
USA | 26 |
Other countries | 11.7 |
World total in 2010 | 176 Mt |
Source: IAEA1
Phosphate rock (phosphorite) is a marine sedimentary rock which contains 18-40% P2O5, as well as some uranium and all its decay products, often 70 to 200 ppmU, and sometimes up to 800 ppm. The main mineral in the phosphate rock is apatite, and most commonly, fluorapatite – Ca5(PO4)3F or Ca10(PO4)6(F,OH)2. This is insoluble, so cannot directly be used as a fertiliser (unless in very acid soils) so must first be processed. This is normally in a wet process phosphoric acid (WPA) plant where it is first dissolved in sulphuric acid. About 2-4% fluorine is usually present. There are about 400 plants using this wet process worldwide, with capacity of some 50 million tonnes P2O5 per year.
Some phosphate deposits – about 4% of total known – are igneous, created by magmatic extrusion as an alkaline chimney. The main mineral is apatite, with some fluorapatite.
When phosphate rock is treated with sulphuric acid in sub stoichiometric quantity, normal superphosphate is formed. If more sulphuric acid is added, a mixture of phosphoric acid and gypsum (calcium sulphate) is obtained. After the gypsum is filtered out, the resultant phosphoric acid can be treated to recover uranium.
The basic reaction is:
Ca3(PO4)2 + 3H2SO4 + 6H2O ==> 2H3PO4 + 3CaSO4.2H2O – exothermic
An improved higher-temperature process produces hemihydrate: CaSO4.1/2H2O
Fluorides need to be controlled as gases and in effluents (HF, fluorosilicic acid) and about half the fluorine reports with the gypsum. In the process a lot of crud is generated, and this was disposed of with gypsum tailings, despite its low-level radioactivity.
After the gypsum precipitation stage, triple superphosphate is obtained by reacting the phosphoric acid with further phosphate rock. Otherwise, various ammonium phosphate fertilizers can be produced by reacting the phosphoric acid with ammonia.
The uranium is normally recovered from the phosphoric acid (H3PO4 – bearing about 28% P2O5) by some form of solvent extraction (SX). Kamorphos is developing a simpler version of this.
PhosEnergy, an ion exchange (IX) process, represents a step-change refinement of the old processes. It was announced in 2009, offering uranium recovery costs of $25-30 /lb U3O8, compared with historical costs of double this.a A demonstration plant was built in Adelaide, South Australia, and shipped to the USA to operate at a US fertilizer producer, where it was commissioned in May 2012. The test campaign with four trials on two feed sources was successful, showing recoveries of over 92% at $20-25/lb operating cost, and with Cameco very fully involved. Full evaluation of the project operation with an engineering study was reported in March 2013, and $18/lb operating cost with capital cost of $156 million for a base case 400 t/yr U3O8 plant were quoted. Cameco reaffirmed its commitment in subscribing a further $4 million.
The process involves taking 27% acid, redox reduction to ferrous iron giving oxidation of uranium, primary IX ,then recycle of acid with improved quality and a secondary IX uranium recovery being much the same as that in Cameco’s US ISL operations, so potential synergy. Bottom line of process is 95% U recovery, no radwaste, $18/lb cost, improved acid quality for main plant, $120 million bolt-on plant cost.
The next phase of PhosEnergy commercialisation is expected to be a continuous on-site demonstration scale operation at the site of an existing phosphate producer. This phase will underpin a Definitive Feasibility Study (DFS) and be the basis for a full-scale commercial facility, which might be constructed and commissioned within three years of the commencement of a DFS.
As well as the PhosEnergy project, Cameco is involved independently through Nukem with CF Industries in developing a facility to recover about 400 tU/yr from Florida phosphates.
In the USA eight plants for the recovery of uranium from phosphoric acid have been built and operated since the 1970s (six in Florida, two in Louisiana). Plants have also been built in Canada, Spain, Belgium (for Moroccan phosphate), Israel, and Taiwan. Brazil is planning a new plant with uranium as co-product with phosphate from 0.08%U ore in igneous rock, to operate from 2012 at 1000 tU/yr. Morocco has by far the largest known resources of uranium in phosphate rock. Egypt reports 42,000 tU in phosphate rocks, at 50-200 ppm U.
The potential amount of uranium able to be recovered from WPA phosphoric acid streams is over 11,000 tonnes U per year (global P2O5 production in 2010 was 33.6 Mt). The economic benefit will be both in the value of the uranium and in reduced regulatory demands on disposal of low-level radioactive wastes arising from the WPA process. Estimated uranium production costs will put the new process in the lowest quartile of new uranium production.
Santa Quiteria and Itataia mines, Brazil
This has reserves of 340 Mt of phosphate containing 140,000 tU at Santa Quiteria and 80,000 tU at Itataia, grading 0.054% U in P2O5, giving 1270 tU/yr from about 2015, 970 tU of this from Itataia.
USA
The country has reserves of 1400 Mt phosphates containing 170,000 tU. At 9.6 Mt/yr P2O5 production, this would yield 2300-2680 tU/yr by-product. Nukem and CF Industries were planning a 430 tU/yr uranium recovery operation at CF's Plant City.
Jordan
The country has reserves of 1500 Mt phosphates containing up to 140,000 tU. At 676,000 t/yr P2O5 production the uranium potential is 135 tU/yr. The government is putting out the Qatrana phosphorites for tender to develop, containing 52 Mt phosphate and 22,000 tU with vanadium.
Egypt
The country has reserves of 100 Mt phosphates containing 40,000 tU.
Tunisia
The country has reserves of 100 Mt phosphates containing 50,000 tU. At 1.6 Mt/yr P2O5 production, this would yield 265 tU/yr by-product.
Morocco
The country has reserves of 50 billion tonnes of phosphates containing 6.9 MtU. At 4.8 Mt/yr P2O5 production, this would yield 960 tU/yr by-product. There is some expectation of 1900 tU/yr production from 2017.
Further Information
Notes
a. The PhosEnergy process is being developed by Uranium Equities Limited (UEQ) through a US registered company, Urtek LLC. Cameco secured rights to earn up to a 73% interest in the technology, and initially paid $12.5 of the $16.5 million required for this to UEQ and a further $4.5 million for the founder's 10%. UEQ subsequently agreed to pay Cameco a share of that 10%, to hold 27% of the rights for the process. Cameco paid a further $4 million in March 2013 to reach 73% share. On the basis of its earlier 70% interest, Cameco agreed to provide funding for a minimum of 50% of UEQ’s portion of capital expenditure for the construction of the first commercial plant, repayable out of earnings. Cameco and UEQ are seeking to enter commercial arrangements with phosphate producers where the process would provide a technical solution for the recovery of uranium from phosphates. The capital required to install the process would be in exchange for off-take from the facility. [Back]References
1. International Atomic Energy Agency, Safety Reports series No. 78, Radiation Protection And Management of NORM Residues in the Phosphate Industry (2013) [Back]General sources
Guzman, ETR et al., Uranium in Phosphate Rock and Derivatives (1995)WISE, Uranium recovery from phosphates
Nuclear Power Reactors
(updated July 2013)
http://world-nuclear.org/info/Nuclear-Fuel-Cycle/Power-Reactors/Nuclear-Power-Reactors/#.UhXG5X-N6So
- Most nuclear electricity is generated using just two kinds of reactors which were developed in the 1950s and improved since.
- New designs are coming forward and some are in operation as the first generation reactors come to the end of their operating lives.
- Around 13% of the world's electricity is produced from nuclear energy, more than from all sources worldwide in 1960.
A nuclear reactor produces and controls the release of energy from
splitting the atoms of certain elements. In a nuclear power reactor, the
energy released is used as heat to make steam to generate electricity.
(In a research reactor the main purpose is to utilise the actual
neutrons produced in the core. In most naval reactors, steam drives a
turbine directly for propulsion.)
The principles for using nuclear power to produce electricity are the
same for most types of reactor. The energy released from continuous
fission of the atoms of the fuel is harnessed as heat in either a gas or
water, and is used to produce steam. The steam is used to drive the
turbines which produce electricity (as in most fossil fuel plants).
The world's first nuclear reactors operated naturally in a uranium
deposit about two billion years ago. These were in rich uranium
orebodies and moderated by percolating rainwater. Those at Oklo in west
Africa, each less than 100 kW thermal, together consumed about six
tonnes of that uranium.
Today, reactors derived from designs originally developed for
propelling submarines and large naval ships generate about 85% of the
world's nuclear electricity. The main design is the pressurised water
reactor (PWR) which has water at over 300°C under pressure in its
primary cooling/heat transfer circuit, and generates steam in a
secondary circuit. The less numerous boiling water reactor (BWR) makes
steam in the primary circuit above the reactor core, at similar
temperatures and pressure. Both types use water as both coolant and
moderator, to slow neutrons. Since water normally boils at 100°C, they
have robust steel pressure vessels or tubes to enable the higher
operating temperature. (Another type uses heavy water, with deuterium
atoms, as moderator. Hence the term ‘light water’ is used to
differentiate.)
Components of a nuclear reactor
There are several components common to most types of reactors:
Fuel. Uranium is the basic fuel. Usually pellets of uranium oxide (UO2) are arranged in tubes to form fuel rods. The rods are arranged into fuel assemblies in the reactor core.*
* In a new reactor with new fuel a neutron source is needed to get the reaction going. Usually this is beryllium mixed with polonium, radium or other alpha-emitter. Alpha particles from the decay cause a release of neutrons from the beryllium as it turns to carbon-12. Restarting a reactor with some used fuel may not require this, as there may be enough neutrons to achieve criticality when control rods are removed.
* In a new reactor with new fuel a neutron source is needed to get the reaction going. Usually this is beryllium mixed with polonium, radium or other alpha-emitter. Alpha particles from the decay cause a release of neutrons from the beryllium as it turns to carbon-12. Restarting a reactor with some used fuel may not require this, as there may be enough neutrons to achieve criticality when control rods are removed.
Moderator. Material in the core which slows down the
neutrons released from fission so that they cause more fission. It is
usually water, but may be heavy water or graphite.
Control rods. These are made with neutron-absorbing
material such as cadmium, hafnium or boron, and are inserted or
withdrawn from the core to control the rate of reaction, or to halt
it.* In some PWR reactors, special control rods are used to enable the
core to sustain a low level of power efficiently. (Secondary control
systems involve other neutron absorbers, usually boron in the coolant –
its concentration can be adjusted over time as the fuel burns up.)
* In fission, most of the neutrons are released promptly, but some are delayed. These are crucial in enabling a chain reacting system (or reactor) to be controllable and to be able to be held precisely critical.
* In fission, most of the neutrons are released promptly, but some are delayed. These are crucial in enabling a chain reacting system (or reactor) to be controllable and to be able to be held precisely critical.
Coolant. A fluid circulating through the core so as
to transfer the heat from it. In light water reactors the water
moderator functions also as primary coolant. Except in BWRs, there is
secondary coolant circuit where the water becomes steam. (See also later
section on primary coolant characteristics)
Pressure vessel or pressure tubes. Usually a robust
steel vessel containing the reactor core and moderator/coolant, but it
may be a series of tubes holding the fuel and conveying the coolant
through the surrounding moderator.
Steam generator.(not
in BWR) Part of the cooling system where the high-pressure primary
coolant bringing heat from the reactor is used to make steam for the
turbine, in a secondary circuit. Essentially a heat exchanger like a
motor car radiator*. Reactors may have up to four 'loops', each with a
steam generator.
* These are large heat exchangers for transferring heat from one fluid to another – here from high-pressure primary circuit in PWR to secondary circuit where water turns to steam. Each structure weighs up to 800 tonnes and contains from 300 to 16,000 tubes about 2 cm diameter for the primary coolant, which is radioactive due to nitrogen-16 (N-16, formed by neutron bombardment of oxygen, with half-life of 7 seconds). The secondary water must flow through the support structures for the tubes. The whole thing needs to be designed so that the tubes don't vibrate and fret, operated so that deposits do not build up to impede the flow, and maintained chemically to avoid corrosion. Tubes which fail and leak are plugged, and surplus capacity is designed to allow for this. Leaks can be detected by monitoring N-16 levels in the steam as it leaves the steam generator.
Containment. The structure around the reactor and
associated steam generators which is designed to protect it from outside
intrusion and to protect those outside from the effects of radiation in
case of any serious malfunction inside. It is typically a metre-thick
concrete and steel structure.
There are several different types of reactors as indicated in the following Table.
Nuclear power plants in commercial operation
Reactor type | Main Countries | Number | GWe | Fuel | Coolant | Moderator |
Pressurised Water Reactor (PWR) |
US, France, Japan, Russia, China
|
271
|
270.4
|
enriched UO2
|
water
|
water
|
---|---|---|---|---|---|---|
Boiling Water Reactor (BWR) |
US, Japan, Sweden
|
84
|
81.2
|
enriched UO2
|
water
|
water
|
Pressurised Heavy Water Reactor 'CANDU' (PHWR) |
Canada
|
48
|
27.1
|
natural UO2
|
heavy water
|
heavy water
|
Gas-cooled Reactor (AGR & Magnox) |
UK
|
17
|
9.6
|
natural U (metal),
enriched UO2 |
CO2
|
graphite
|
Light Water Graphite Reactor (RBMK & EGP) |
Russia
|
11 + 4
|
10.4
|
enriched UO2
|
water
|
graphite
|
Fast Neutron Reactor (FBR) |
Russia
|
1
|
0.6
|
PuO2 and UO2
|
liquid sodium
|
none
|
TOTAL | 436 | 399.3 |
GWe = capacity in thousands of megawatts (gross)
Source: Nuclear Engineering International Handbook 2011, updated to 1/1/12
For reactors under construction: see paper Plans for New Reactors Worldwide.
Source: Nuclear Engineering International Handbook 2011, updated to 1/1/12
For reactors under construction: see paper Plans for New Reactors Worldwide.
Fuelling a nuclear power reactor
Most reactors need to be shut down for refuelling, so that the
pressure vessel can be opened up. In this case refuelling is at
intervals of 1-2 years, when a quarter to a third of the fuel assemblies
are replaced with fresh ones. The CANDU and RBMK types have pressure
tubes (rather than a pressure vessel enclosing the reactor core) and can
be refuelled under load by disconnecting individual pressure tubes.
If graphite or heavy water is used as moderator, it is possible to
run a power reactor on natural instead of enriched uranium. Natural
uranium has the same elemental composition as when it was mined (0.7%
U-235, over 99.2% U-238), enriched uranium has had the proportion of the
fissile isotope (U-235) increased by a process called enrichment,
commonly to 3.5 - 5.0%. In this case the moderator can be ordinary
water, and such reactors are collectively called light water reactors.
Because the light water absorbs neutrons as well as slowing them, it is
less efficient as a moderator than heavy water or graphite.
During operation, some of the U-238 is changed to plutonium, and Pu-239 ends up providing about one third of the energy from the fuel.
In most reactors the fuel is ceramic uranium oxide (UO2
with a melting point of 2800°C) and most is enriched. The fuel pellets
(usually about 1 cm diameter and 1.5 cm long) are typically arranged in a
long zirconium alloy (zircaloy) tube to form a fuel rod, the zirconium
being hard, corrosion-resistant and permeable to neutrons.* Numerous
rods form a fuel assembly, which is an open lattice and can be lifted
into and out of the reactor core. In the most common reactors these are
about 3.5 to 4 metres long.
*Zirconium is an important mineral for nuclear power, where it finds its main use. It is therefore subject to controls on trading. It is normally contaminated with hafnium, a neutron absorber, so very pure 'nuclear grade' Zr is used to make the zircaloy, which is about 98% Zr plus tin, iron, chromium and sometimes nickel to enhance its strength.
*Zirconium is an important mineral for nuclear power, where it finds its main use. It is therefore subject to controls on trading. It is normally contaminated with hafnium, a neutron absorber, so very pure 'nuclear grade' Zr is used to make the zircaloy, which is about 98% Zr plus tin, iron, chromium and sometimes nickel to enhance its strength.
Burnable poisons are often used (especially in BWR) in fuel or
coolant to even out the performance of the reactor over time from fresh
fuel being loaded to refuelling. These are neutron absorbers which decay
under neutron exposure, compensating for the progressive build up of
neutron absorbers in the fuel as it is burned. The best known is
gadolinium, which is a vital ingredient of fuel in naval reactors where
installing fresh fuel is very inconvenient, so reactors are designed to
run more than a decade between refuellings.
The power rating of a nuclear power reactor
Nuclear power plant reactor power outputs are quoted in three ways:
- Thermal MWt, which depends on the design of the actual nuclear reactor itself, and relates to the quantity and quality of the steam it produces.
- Gross electrical MWe indicates the power produced by the attached steam turbine and generator, and also takes into account the ambient temperature for the condenser circuit (cooler means more electric power, warmer means less). Rated gross power assumes certain conditions with both.
- Net electrical MWe, which is the power available to be sent out from the plant to the grid, after deducting the electrical power needed to run the reactor (cooling and feed-water pumps, etc.) and the rest of the plant.*
* Net electrical MWe and gross MWe vary
slightly from summer to winter, so normally the lower summer figure, or
an average figure, is used. If the summer figure is quoted plants may
show a capacity factor greater than 100% in cooler times. Some design
options, such as powering the main large feed-water pumps with electric
motors (as in EPR) rather than steam turbines (taking steam before it
gets to the main turbine-generator), explains some gross to net
differences between different reactor types. The EPR has a relatively
large drop from gross to net MWe for this reason.

The relationship between these is expressed in two ways:
- Thermal efficiency %, the ratio of gross MWe to thermal MW. This relates to the difference in temperature between the steam from the reactor and the cooling water. It is often 33-37%.
- Net efficiency %, the ratio of net MWe achieved to thermal MW. This is a little lower, and allows for plant usage.
In WNA papers and figures and WNN items, generally net MWe is used
for operating plants, and gross MWe for those under construction or
planned/proposed.
Pressurised Water Reactor (PWR)
This is the most common type, with over 230 in use for power
generation and several hundred more employed for naval propulsion. The
design of PWRs originated as a submarine power plant.
PWRs use ordinary water as both coolant and moderator. The design is
distinguished by having a primary cooling circuit which flows through
the core of the reactor under very high pressure, and a secondary
circuit in which steam is generated to drive the turbine. In Russia
these are known as VVER types - water-moderated and -cooled.

A PWR has fuel assemblies of 200-300 rods each, arranged vertically
in the core, and a large reactor would have about 150-250 fuel
assemblies with 80-100 tonnes of uranium.
Water in the reactor core reaches about 325°C, hence it must be kept
under about 150 times atmospheric pressure to prevent it boiling.
Pressure is maintained by steam in a pressuriser (see diagram). In the
primary cooling circuit the water is also the moderator, and if any of
it turned to steam the fission reaction would slow down. This negative
feedback effect is one of the safety features of the type. The secondary
shutdown system involves adding boron to the primary circuit.
The secondary circuit is under less pressure and the water here boils
in the heat exchangers which are thus steam generators. The steam
drives the turbine to produce electricity, and is then condensed and
returned to the heat exchangers in contact with the primary circuit.
Boiling Water Reactor (BWR)
This design has many similarities to the PWR, except that there is
only a single circuit in which the water is at lower pressure (about 75
times atmospheric pressure) so that it boils in the core at about 285°C.
The reactor is designed to operate with 12-15% of the water in the top
part of the core as steam, and hence with less moderating effect and
thus efficiency there. BWR units can operate in load-following mode
more readily then PWRs.
The steam passes through drier plates (steam separators) above the
core and then directly to the turbines, which are thus part of the
reactor circuit. Since the water around the core of a reactor is always
contaminated with traces of radionuclides, it means that the turbine
must be shielded and radiological protection provided during
maintenance. The cost of this tends to balance the savings due to the
simpler design. Most of the radioactivity in the water is very
short-lived*, so the turbine hall can be entered soon after the reactor
is shut down.
* mostly N-16, with a 7 second half-life
A BWR fuel assembly comprises 90-100 fuel rods, and there are up to
750 assemblies in a reactor core, holding up to 140 tonnes of uranium.
The secondary control system involves restricting water flow through the
core so that more steam in the top part reduces moderation.

Pressurised Heavy Water Reactor (PHWR or CANDU)
The PHWR reactor design has been developed since the 1950s in Canada
as the CANDU, and more recently also in India. PHWRs generally use
natural uranium (0.7% U-235) oxide as fuel, hence needs a more efficient
moderator, in this case heavy water (D2O).** The PHWR produces more energy per kg of mined uranium than other designs.
** with the CANDU system, the moderator is enriched (ie water) rather than the fuel, - a cost trade-off.
The moderator is in a large tank called a calandria, penetrated by
several hundred horizontal pressure tubes which form channels for the
fuel, cooled by a flow of heavy water under high pressure in the primary
cooling circuit, reaching 290°C. As in the PWR, the primary coolant
generates steam in a secondary circuit to drive the turbines. The
pressure tube design means that the reactor can be refuelled
progressively without shutting down, by isolating individual pressure
tubes from the cooling circuit.

A CANDU fuel assembly consists of a bundle of 37 half metre long fuel
rods (ceramic fuel pellets in zircaloy tubes) plus a support structure,
with 12 bundles lying end to end in a fuel channel. Control rods
penetrate the calandria vertically, and a secondary shutdown system
involves adding gadolinium to the moderator. The heavy water moderator
circulating through the body of the calandria vessel also yields some
heat (though this circuit is not shown on the diagram above).
Newer PHWR designs such as the Advanced Candu Reactor (ACR) have light water cooling and slightly-enriched fuel.
CANDU reactors can readily be run on recycled uranium from
reprocessing LWR used fuel, or a blend of this and depleted uranium left
over from enrichment plants. About 4000 MWe of PWR can then fuel 1000
MWe of CANDU capacity, with addition of depleted uranium. Thorium may
also be used in fuel.
Advanced Gas-cooled Reactor (AGR)
These are the second generation of British gas-cooled reactors, using
graphite moderator and carbon dioxide as primary coolant. The fuel is
uranium oxide pellets, enriched to 2.5-3.5%, in stainless steel tubes.
The carbon dioxide circulates through the core, reaching 650°C and then
past steam generator tubes outside it, but still inside the concrete and
steel pressure vessel (hence 'integral' design). Control rods penetrate
the moderator and a secondary shutdown system involves injecting
nitrogen to the coolant.

The AGR was developed from the Magnox reactor, also graphite moderated and CO2
cooled, and one of these is still operating in UK to late 2014. They
use natural uranium fuel in metal form. Secondary coolant is water.
Light water graphite-moderated reactor (RBMK)
This is a Soviet design, developed from plutonium production
reactors. It employs long (7 metre) vertical pressure tubes running
through graphite moderator, and is cooled by water, which is allowed to
boil in the core at 290°C, much as in a BWR. Fuel is low-enriched
uranium oxide made up into fuel assemblies 3.5 metres long. With
moderation largely due to the fixed graphite, excess boiling simply
reduces the cooling and neutron absorbtion without inhibiting the
fission reaction, and a positive feedback problem can arise, which is
why they have never been built outside the Soviet Union. See appendix on
RBMK Reactors for more detail.
Advanced reactors
Several generations of reactors are commonly distinguished.
Generation I reactors were developed in 1950-60s and only one is still
running today. They mostly used natural uranium fuel and used graphite
as moderator. Generation II reactors are typified by the present US
fleet and most in operation elsewhere. They typically use enriched
uranium fuel and are mostly cooled and moderated by water. Generation
III are the Advanced Reactors evolved from these, the first few of which
are in operation in Japan and others are under construction and ready
to be ordered. They are developments of the second generation with
enhanced safety. There is no clear distinction Gen II to Gen III.
Generation IV designs are still on the drawing board and will not be
operational before 2020 at the earliest, probably later. They will tend
to have closed fuel cycles and burn the long-lived actinides now forming
part of spent fuel, so that fission products are the only high-level
waste. Of seven designs under development, 4 or 5 will be fast neutron
reactors. Four will use fluoride or liquid metal coolants, hence operate
at low pressure. Two will be gas-cooled. Most will run at much higher
temperatures than today’s water-cooled reactors. See Generation IV Reactors paper.
More than a dozen (Generation III) advanced reactor
designs are in various stages of development. Some are evolutionary
from the PWR, BWR and CANDU designs above, some are more radical
departures. The former include the Advanced Boiling Water Reactor, a few
of which are now operating with others under construction. The
best-known radical new design has the fuel as large 'pebbles' and uses
helium as coolant, at very high temperature, possibly to drive a turbine
directly.
Considering the closed fuel cycle, Generation 1-3 reactors recycle
plutonium (and possibly uranium), while Generation IV are expected to
have full actinide recycle.
Fast neutron reactors (FNR)
Some reactors (only one in commercial service) do not have a
moderator and utilise fast neutrons, generating power from plutonium
while making more of it from the U-238 isotope in or around the fuel.
While they get more than 60 times as much energy from the original
uranium compared with the normal reactors, they are expensive to build.
Further development of them is likely in the next decade, and the main
designs expected to be built in two decades are FNRs. If they are
configured to produce more fissile material (plutonium) than they
consume they are called Fast Breeder Reactors (FBR). See also Fast Neutron Reactors and Small Reactors papers.
Floating nuclear power plants
Apart from over 200 nuclear reactors powering various kinds of ships,
Rosatom in Russia has set up a subsidiary to supply floating nuclear
power plants ranging in size from 70 to 600 MWe. These will be mounted
in pairs on a large barge, which will be permanently moored where it is
needed to supply power and possibly some desalination to a shore
settlement or industrial complex. The first has two 40 MWe reactors
based on those in icebreakers and will operate at a remote site in
Siberia. Electricity cost is expected to be much lower than from present
alternatives.
The Russian KLT-40S is a reactor well proven in icebreakers and now
proposed for wider use in desalination and, on barges, for remote area
power supply. Here a 150 MWt unit produces 35 MWe (gross) as well as up
to 35 MW of heat for desalination or district heating. These are
designed to run 3-4 years between refuelling and it is envisaged that
they will be operated in pairs to allow for outages, with on-board
refuelling capability and used fuel storage. At the end of a 12-year
operating cycle the whole plant is taken to a central facility for
2-year overhaul and removal of used fuel, before being returned to
service. Two units will be mounted on a 21,000 tonne barge. A larger
Russian factory-built and barge-mounted reactor is the VBER-150, of 350
MW thermal, 110 MWe. The larger VBER-300 PWR is a 325 MWe unit,
originally envisaged in pairs as a floating nuclear power plant,
displacing 49,000 tonnes. As a cogeneration plant it is rated at 200 MWe
and 1900 GJ/hr. See also Nuclear Power in Russia paper.
Lifetime of nuclear reactors
Most of today's nuclear plants which were originally designed for 30
or 40-year operating lives. However, with major investments in systems,
structures and components lives can be extended, and in several
countries there are active programs to extend operating lives. In the
USA most of the more than one hundred reactors are expected to be
granted licence extensions from 40 to 60 years. This justifies
significant capital expenditure in upgrading systems and components,
including building in extra performance margins.
Some components simply wear out, corrode or degrade to a low level of
efficiency. These need to be replaced. Steam generators are the most
prominent and expensive of these, and many have been replaced after
about 30 years where the reactor otherwise has the prospect of running
for 60 years. This is essentially an economic decision. Lesser
components are more straightforward to replace as they age. In Candu
reactors, pressure tube replacement has been undertaken on some plants
after about 30 years operation.
A second issue is that of obsolescence. For instance, older reactors
have analogue instrument and control systems. Thirdly, the properties of
materials may degrade with age, particularly with heat and neutron
irradiation. In respect to all these aspects, investment is needed to
maintain reliability and safety. Also, periodic safety reviews are
undertaken on older plants in line with international safety conventions
and principles to ensure that safety margins are maintained.
Another important issue is knowledge management (KM) over the full
lifecycle from design, through construction and operation to
decommissioning for reactors and other facilities. This may span a
century and involve several countries, and involve a succession of
companies. The plant lifespan will cover several generations of
engineers. Data needs to be transferable across several generations of
software and IT hardware, as well as being shared with other operators
of similar plants.* Significant modifications may be made to the design
over the life of the plant, so original documentation is not
sufficient, and loss of design base knowledge can have huge implications
(eg Pickering A and Bruce A in Ontario). Knowledge management is often a
shared responsibility and is essential for effective decision-making
and the achievement of plant safety and economics.
* ISO15926 covers portability and interoperability for lifecycle open data standard. Also EPRI in 2013 published Advanced Nuclear Technology: New Nuclear Power Plant Information Handover Guide.
See also section on Ageing, in Safety of Nuclear Power Reactors paper.
Load-following capacity
Nuclear power plants are essentially base-load generators, running
continuously. This is because their power output cannot readily be
ramped up and down on a daily and weekly basis, and in this respect they
are similar to most coal-fired plants. (It is also uneconomic to run
them at less than full capacity, since they are expensive to build but
cheap to run.) However, in some situations it is necessary to vary the
output according to daily and weekly load cycles on a regular basis, for
instance in France, where there is a very high reliance on nuclear
power.
While BWRs can be made to follow loads reasonably easily without
burning the core unevenly, this is not as readily achieved in a PWR. The
ability of a PWR to run at less than full power for much of the time
depends on whether it is in the early part of its 18 to 24-month
refueling cycle or late in it, and whether it is designed with special
control rods which diminish power levels throughout the core without
shutting it down. Thus, though the ability on any individual PWR reactor
to run on a sustained basis at low power decreases markedly as it
progresses through the refueling cycle, there is considerable scope for
running a fleet of reactors in load-following mode. See further
information in the Nuclear Power in France paper.
As fast neutron reactors become established in future years, their ability to load-follow will be a benefit.
Primary coolants
The advent of some of the designs mentioned above provides
opportunity to review the various primary coolants used in nuclear
reactors. There is a wide variety – gas, water, light metal, heavy metal
and salt:
Water or heavy water must
be maintained at very high pressure (1000-2200 psi, 7-15 MPa) to enable
it to function above 100°C, as in present reactors. This has a major
influence on reactor engineering. However, supercritical water around 25
MPa can give 45% thermal efficiency – as at some fossil-fuel power
plants today with outlet temperatures of 600°C, and at ultra
supercritical levels (30+ MPa) 50% may be attained.
Water cooling of steam condensers is fairly standard in all power
plants, because it works very well, it is relatively inexpensive, and
there is a huge experience base. Water is a lot more effective than air
for removing heat.
Helium must be
used at similar pressure (1000-2000 psi, 7-14 MPa) to maintain
sufficient density for efficient operation. Again, there are engineering
implications, but it can be used in the Brayton cycle to drive a
turbine directly.
Carbon dioxide
was used in early British reactors and their AGRs which operate at much
higher temperatures than light water reactors. It is denser than helium
and thus likely to give better thermal conversion efficiency. There is
now interest in supercritical CO2 for the Brayton cycle.
Sodium, as
normally used in fast neutron reactors at around 550ºC, melts at 98°C
and boils at 883°C at atmospheric pressure, so despite the need to keep
it dry the engineering required to contain it is relatively modest. It
has high thermal conductivity. However, normally water/steam is used in
the secondary circuit to drive a turbine (Rankine cycle) at lower
thermal efficiency than the Brayton cycle. In some designs sodium is in a
secondary circuit to steam generators. Sodium does not corrode the
metals used in the fuel cladding or primary circuit, nor the fuel itself
if there is cladding damage.
Lead or lead-bismuth eutectic
in fast neutron reactors are capable of higher temperature operation.
They are transparent to neutrons, aiding efficiency, and since they do
not react with water the heat exchanger interface is safer. They do not
burn when exposed to air. However, they are corrosive of fuel cladding
and steels, which originally limited temperatures to 550°C. With today's
materials 650°C can be reached, and in future 700°C is in sight, using
oxide dispersion-strengthened steels. A problem is that Pb-Bi yields
toxic polonium (Po-210) activation products. Pb-Bi melts at a relatively
low 125°C (hence eutectic) and boils at 1670°C, Pb melts at 327°C and
boils at 1737°C but is very much more abundant and cheaper to produce
than bismuth, hence is envisaged for large-scale use in the future,
though freezing must be prevented. The development of nuclear power
based on Pb-Bi cooled fast neutron reactors is likely to be limited to a
total of 50-100 GWe, basically for small reactors in remote places. In
1998 Russia declassified a lot of research information derived from its
experience with submarine reactors, and US interest in using Pb or Pb-Bi
for small reactors has increased subsequently. The Gen4 Module
(Hyperion) reactor will use lead-bismuth eutectic which is 45% Pb, 55%
Bi. A secondary circuit generating steam is likely.
Molten fluoride salt
boils at 1400°C at atmospheric pressure, so allows several options for
use of the heat, including using helium in a secondary Brayton cycle
circuit with thermal efficiencies of 48% at 750°C to 59% at 1000°C, or
manufacture of hydrogen. Fluoride salts have very low vapour pressure
even at red heat, have reasonably good heat transfer properties, are not
damaged by radiation, do not react violently with air or water, and are
inert to some common structural metals.
Low-pressure liquid coolants allow all their heat to be delivered at
high temperatures, since the temperature drop in heat exchangers is less
than with gas coolants. Also, with a good margin between operating and
boiling temperatures, passive cooling for decay heat is readily
achieved.
The removal of passive decay heat is a vital feature of primary
cooling systems, beyond heat transfer to do work. When the fission
process stops, fission product decay continues and a substantial amount
of heat is added to the core. At the moment of shutdown, this is about
6.5% of the full power level, but after an hour it drops to about 1.5%
as the short-lived fission products decay. After a day, the decay heat
falls to 0.4%, and after a week it will be only 0.2%. This heat could
melt the core of a light water reactor unless it is reliably dissipated,
as shown in 2011 at Fukushima, where about 1.5% of the heat was being
generated when the tsunami disabled the cooling. In passive systems,
some kind of convection flow is relied upon.

Top AHTR line is potential, lower one practical today. See also paper on Cooling Power Plants.
There is some radioactivity in the cooling water flowing through the
core of a water-cooled reactor, due mainly to the activation product
nitrogen-16, formed by neutron capture from oxygen. N-16 has a half-life
on only 7 seconds but produces high-energy gamma radiation during
decay. It is the reason that access to a BWR turbine hall is restricted
during actual operation.
Nuclear reactors for process heat
Producing steam to drive a turbine and generator is relatively easy,
and a light water reactor running at 350°C does this readily. As the
above section and Figure show, other types of reactor are required for
higher temperatures. A 2010 US Department of Energy document quotes
500°C for a liquid metal cooled reactor (FNR), 860°C for a molten salt
reactor (MSR), and 950°C for a high temperature gas-cooled reactor
(HTR). Lower-temperature reactors can be used with supplemental gas
heating to reach higher temperatures, though employing an LWR would not
be practical or economic. The DOE said that high reactor outlet
temperatures in the range 750 to 950°C were required to satisfy all end
user requirements evaluated to date for the Next Generation Nuclear
Plant.
Primitive reactors
The world's oldest known nuclear reactors operated at what is now
Oklo in Gabon, West Africa. About 2 billion years ago, at least 17
natural nuclear reactors achieved criticality in a rich deposit of
uranium ore. Each operated intermittently at about 20 kW thermal, the
reaction ceasing whenever the water turned to steam so that it ceased to
function as moderator. At that time the concentration of U-235 in all
natural uranium was 3.7 percent instead of 0.7 percent as at present.
(U-235 decays much faster than U-238, whose half-life is about the same
as the age of the Earth.) These natural chain reactions, started
spontaneously by the presence of water acting as a moderator, continued
overall for about 2 million years before finally dying away.
During this long reaction period about 5.4 tonnes of fission products
as well as 1.5 tonnes of plutonium together with other transuranic
elements were generated in the orebody. The initial radioactive products
have long since decayed into stable elements but close study of the
amount and location of these has shown that there was little movement of
radioactive wastes during and after the nuclear reactions. Plutonium
and the other transuranics remained immobile.
Sources:
Wilson, P.D., 1996, The Nuclear Fuel Cycle, OUP.
Wilson, P.D., 1996, The Nuclear Fuel Cycle, OUP.
Tidak ada komentar:
Posting Komentar